Mechanisms of Developmental Biology Series: Essence of All
- Gülce Tekin
- Sep 18, 2023
- 18 min read
Updated: Sep 11, 2024
Foreword
The progression from a single-cell entity to a multicellular organism entails a series of intricate stages. This intricate journey involves the orchestrated activities of stem cells and the process of differentiation, which collectively constitute the life cycle of complex, multicellular organisms. The domain of developmental biology transcends human boundaries, encompassing studies of animals and plants alike, underscoring its extensive purview. The pursuit of unraveling the intricate mechanisms governing organismal development not only illuminates the origins of life but also provides invaluable insights into the molecular and cellular underpinnings of development across diverse species. The curriculum of this developmental biology series serves as an illuminating beacon, shedding light on the myriad manifestations of life on our planet. To gain a more profound comprehension of developmental processes, this series will traverse multiple interdisciplinary avenues, including embryology, medical science, and evolutionary biology. Through this holistic approach, we endeavor to uncover the underlying principles governing the diverse tapestry of life forms on Earth.
This series will be divided into the following sections:
1. Mechanisms of Developmental Biology Series: Essence of All
6. Mechanisms of Developmental Biology Series: Cutting-edge Techniques in Developmental Biology
Mechanisms of Developmental Biology Series: Essence of All
For a multicellular organism to be formed, the process of development is fundamental. The development of organisms continues throughout their lifespan, contributing to both cellular diversity and passage of traits from one generation to another. Developmental biology concentrates on how different organisms go through the process of development to give rise to a fertile organism from a single cell. New-fashioned approaches for interpreting the developmental process of organisms include paying attention to how cellular growth and differentiation contribute to the journey of organismal development. In this article, the basic concepts of modern developmental biology will be explored by giving detailed information about the processes that arise throughout the developmental process. While doing so, main terminologies such as stem cells, regeneration, and plasticity will be explained. In addition, model organisms such as Drosophila melanogaster (fruit fly), Caenorhabditis elegans (roundworm), Danio rerio (zebrafish), Mus musculus (mouse), Arabidopsis thaliana (thale cress), and Xenopus laevis (African clawed frog) will be discussed in regard to their potential use in developmental biology research to emphasize the essential points of stem cell research for future innovations.
Potency: Stem Cells and Their Ability to Differentiate
Stem cells are the forerunners of other cell types within an organism. They can be distinguished from other cells by their ability to undergo self-renewal to give rise to more cells and differentiate. The ability of a stem cell to differentiate into other cell types can be named as potency (He et al., 2009). The potency of a cell can differ depending on the type of cells it can give rise to, namely, the more types of cells a cell differentiates into, the higher its potency. The concept of potency can further be divided into sub-categories depending on the “ability” of the stem cells' differentiation:
Totipotency can be defined as when a stem cell has the capacity to procreate the whole pool of differentiated cells, either extra embryonic or embryonic tissues, within the organism. Their potential is enough for them to give rise to a complete organism.
Pluripotency appertain to stem cells that can give rise to different kinds of primary germ layers that are established in the course of embryonic development; endoderm, mesoderm, or ectoderm. Nevertheless, they lack the ability to generate extra-embryonic tissues such as the placenta, so the generation of a complete organism is not possible (Díez Villanueva et al., 2012).
Multipotency refers to cell types that can differentiate into a limited range of cell types that are of the same tissue of origin.
Oligopotency means that a stem cell can give rise to a few cell types.
Unipotency refers to stem cells that can lead to the formation of a single type of cell or tissue, and their reproductive capability is unlimited.

Stem Cells and Their Origins
Classification of stem cells based on their source of collection can be divided into five categories: adult, embryonic, fetal, perinatal, and induced pluripotent stem cells. Adult stem cells can be further divided regarding their origin as neural, mesenchymal, hematopoietic, or epithelial stem cells (these classifications may differ from source to source). Similarly, perinatal stem cells can be classified into three subcategories, depending on the types of extra-embryonic structures they are derived from, such as the amniotic fluid, the placenta, and the umbilical cord. Fetal stem cells, on the other hand, are the ones that can result in the formation of organ systems and can be categorized as hematopoietic, mesenchymal, and neural crest stem cells (Bagheri-Mohammadi, 2021).
1) Adult Stem Cells
Adult stem cells (ASCs, or somatic stem cells) are undifferentiated and found in the characteristic differentiated tissues of the human body. For instance, neural stem cells can give rise to different types of brain cells, but not to skin cells, due to their multipotency. As all types of stem cells, they can regenerate and replenish tissue that needs to be replaced which is either too old or damaged. Neural ASCs (NSCs) are the progenitors of brain tissue and result in the formation of the nervous system. These stem cells are localized to specific regions in adult brains. Three of the major neural cells that arise from neural adult stem cells include neurons, astrocytes, and oligodendrocytes (Zhao & Moore, 2018). Mesenchymal ASCs (MSCs) can be retrieved from pericytes (perivascular cells that are present along the capillary intervals) and isolation can be performed from nearly all types of tissue found in the body. They can synthesize bioactive molecules and contribute to the immune system by protecting the damaged sites of the tissue from intruders and providing site-specific tissue regeneration (Caplan, 2015). Hematopoietic stem cells (HSCs, or blood stem cells) are able to differentiate into multiple kinds of mature blood cell types (Hawley& Hawley, 2006), and contribute to blood homeostasis throughout the lifespan of individuals. Epithelial stem cells (ESCs) are responsible for the maintenance of epithelia throughout the body of the individual as well as facilitating wound healing (Blanpain et al., 2021). These stem cells have the ability to renew and differentiate into various tissues according to the function they are attained to.
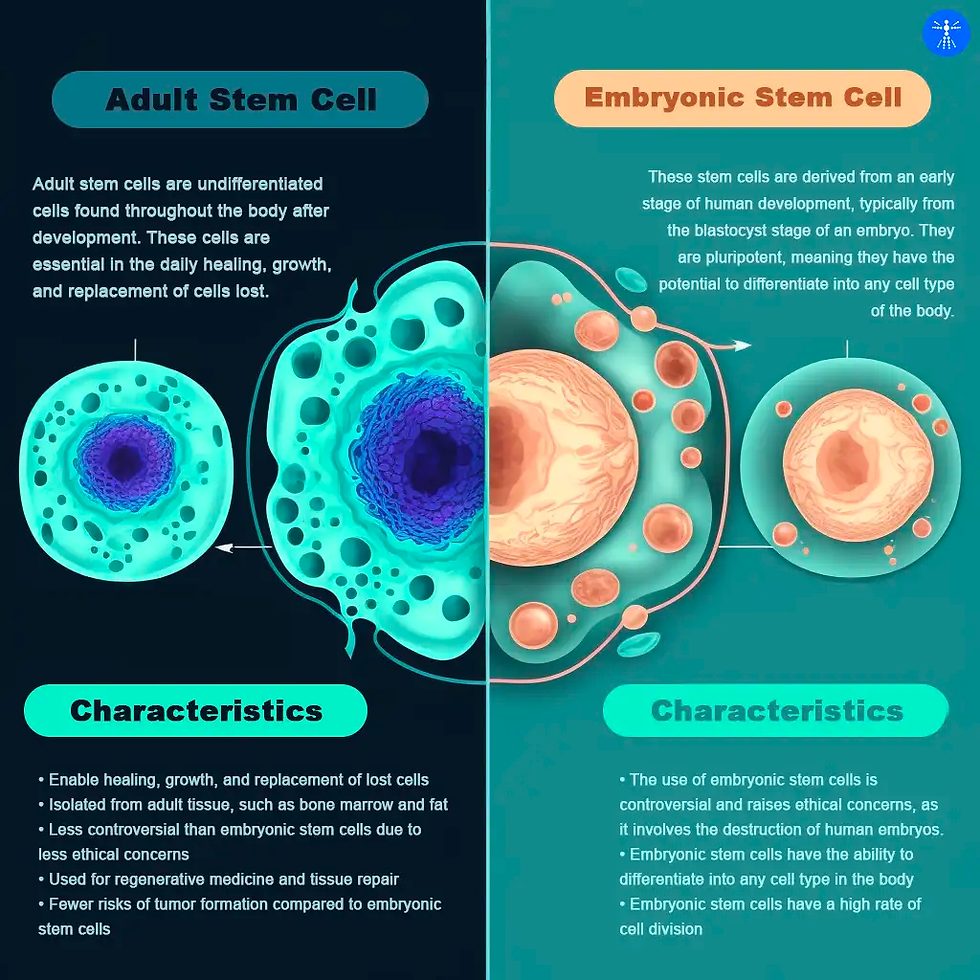
2) Embryonic Stem Cells
The inner side of the blastocyst is the region where embryonic stem cells reside during the first week after fertilization. This is followed by their disappearance in order to form the embryonic tissue layers (National Academies Press, 2002). These cells are pluripotent, meaning that they can form all of the embryonic tissues via differentiation. In the late 90’s, the successful isolation of human embryonic stem cells led to new discoveries and treatment options in the field of developmental biology. So, these cells can be maintained in the laboratory under sufficient conditions and will still keep their pluripotency. Although the research was proceeding relatively slowly due to ethical concerns of society, embryonic stem cell research continued to search for new regenerative medicine options (Cyranoski, 2018).
3) Fetal Stem Cells
Fetal stem cells emerge eight weeks after fertilization, as the fetus forms. They can be obtained from fetal blood, bone marrow, and other tissues of the fetus. They are slightly advantageous to be used in research since they raise fewer ethical concerns and have immense differentiation potential, higher than adult stem cells (O’Donoghue, 2004). Hematopoiesis is essential for the fetus to continue its life. Throughout its development, the system develops into further elements such as erythrocytes and lymphocytes, via the action of hematopoietic fetal HSCs (Waas, Maillard, 2017). Fetal mesenchymal stem cells (fMSC) are observed to acquire immunosuppressive, anti-inflammatory, and proliferative properties. Lastly, neural crest stem cells (NCSCs), that derive pigmentation and facial skeleton (Brenner-Fraser, 1994), can be derived from the neural tube, and then move to specified regions in order to differentiate and form the peripheral nervous system. These remain dormant in the adult period of the individual.
4) Perinatal Stem Cells
Perinatal stem cells can be isolated from the amniotic fluid, the placenta, and the umbilical cord. The reason why these cells exhibit valuable therapy potential is that the placenta contains a high number of cells that are not too exposed to infections, hence, the chances of disease transmission can be relatively low (Torre& Flores, 2020).
5) Induced Pluripotent Stem Cells
In 2006, the laboratory of a Japanese researcher, the Yamanaka lab observed the first induced pluripotent stem cell (iPS) line from mouse fibroblasts. These cells are pluripotent adult stem cells that can be reprogrammed by scientists to become embryonic stem cells. This can be achieved by the forced expression of specific transcription factors within the genome of the somatic cell line. iPS cells promise an effective approach since they acquire the definitive properties of embryonic stem cells (Ye et al., 2013). The fact that somatic stem cells can be reprogrammed into cells that have similar properties to embryonic stem cells has been yet an important discovery in respective stem-cell therapy and regenerative medicine. Therefore, stem cells that have forced pluripotency can be generated from ASCs to give rise to iPS cells and this phenomenon would disqualify the ethical concerns raised by ESC research.
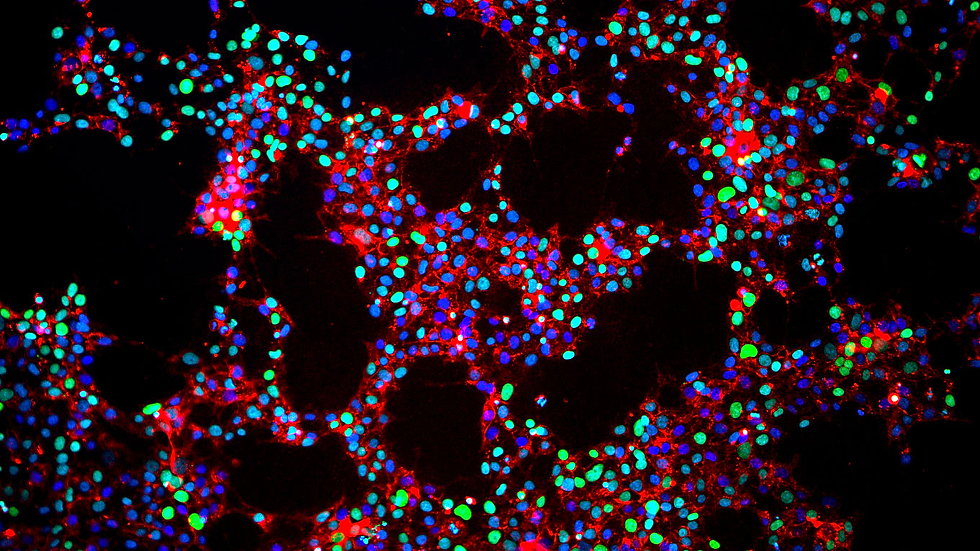
The Stem Cell Niche
The stem cell niche can be defined as the microenvironment of the stem cells that remains dynamic and provides support to stem cells. Research shows that the fate of stem cells, or, in other words, which cell type the stem cell will differentiate into in the following stages of adulthood, is dependent on the communication between stem cells and their environment. The niche of a stem cell communicates with the original population of stem cells via molecular signals, soluble factors, or extracellular proteins. The residence of stem cells is also important when the differentiation and characterization of stem cells are to be addressed. Examples of stem cell niche elements include hormones, endothelial cells, macrophages, chemokines, or transporters (Pennings et al., 2018). Cross-talk within stem cell niches is possible and plays an essential role in the course of stem cell characterization. Recent studies in this field prove that these stem cell niches can be regulated and organized for further understanding of the phenomena.
Morphogenesis
Morphogenesis is the process which leads to cells, organisms, and tissues to develop into their final shapes. The shaping of the final form is achieved by differentiation, depending on the fate of the cells. Morphogenesis can be regulated by factors that are either chemical or mechanical. Among the critical players in morphogenesis are morphogens, which are signaling molecules capable of influencing the internal processes, behaviors, and movements of cells. A cell’s response to morphogens can change depending on their concentration within the environment they are found in. Morphogens work via signaling cascades, by binding to specific receptors and inducing responses within the cell. For example, transcription factors can bind to specific parts of the DNA in order to enhance or inhibit the transcription of several genes, causing a change in the composition of the proteome as well (Gurdon &Bourillot, 2001). So, the cell learns its final position by interpreting the gradient of an extracellular signal factor and develops its fate in regard to that information.
Regeneration
Cell regeneration refers to the process of replacement or restoration of the damaged tissue within an organism. The ability to regenerate can be explained by processes such as scar formation, bone healing, skin, and hair regrowth etc. Examples of organisms with regeneration capability include axolotls, and Mexican salamanders, which are able to regenerate most of their body parts if needed (Seifert& Muneoka, 2018). Similarly, starfish being able to generate another arm when one is lost can also be considered as an example of regeneration of the tissue. Homeostatic regeneration, therefore, refers to these kinds of changes that undergo replacement during the course of tissue homeostasis until the cells are not able to facilitate regeneration.

Different organisms regenerate in different ways. Some animal species generate blastema, rapidly dividing cells, to create the lost parts of the body whereas humans and other animals perform compensatory hypertrophy in which the remaining part of the organ grows back to its original size and compensates for the function. This process is facilitated by the presence of stem cells, however, the capability of regeneration declines as the organism ages.
Model Organisms in Developmental Biology
The twentieth century remains the era in which the concept of model organisms has been investigated profoundly after the works of Mendel and Darwin. Multicellular organisms that are shown to have significance in regard to developmental processes gained insight within the course of these investigations. These model organisms are studied in detail due to the promising features of homology, generation time, and fruitfulness (Irion & Nüsslein-Volhard, 2022). With the newly discovered genetic techniques such as gene editing using CRISPR-Cas mechanisms, these model organisms have shown to be at the heart of genetic research.
Drosophila melanogaster
In the 21st century, in order to study genetics in laboratory conditions, Thomas H. Morgan decided to work with fruit flies due to their easy maintenance. These organisms are fairly easy to be kept in small containers and require little effort to generate new lines. Following the numerous scientific approaches using Drosophila melanogaster as model organisms to study genetics, scientists were successfully able to sequence the whole genome of the flies consisting of five chromosomes (Wolf& Rockmann, 2008). Deficiencies in chromosomes and the location of genes were identified to be visualized in polytene chromosomes of D. melanogaster larvae, providing insights into genetic mapping.
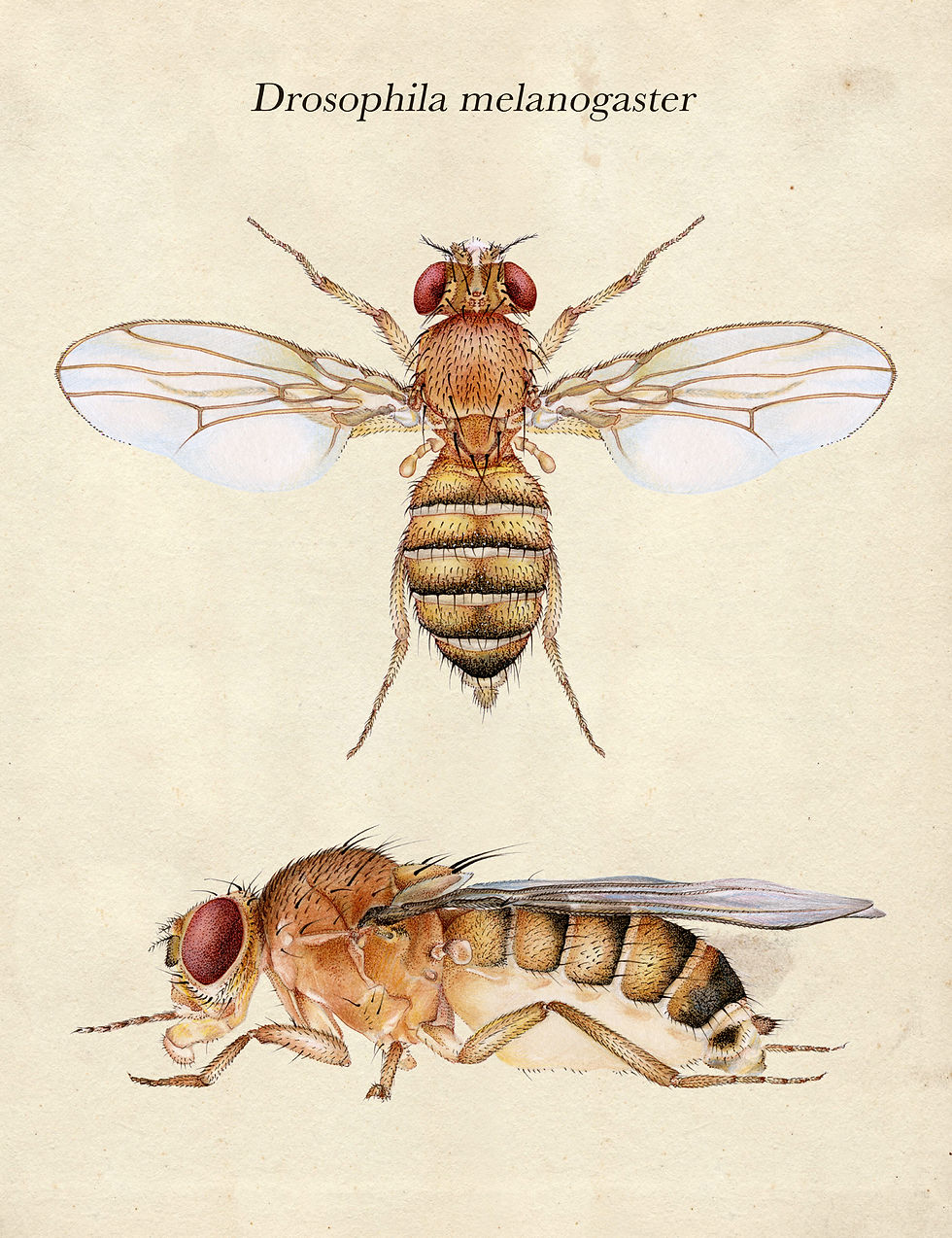
The generation of fruit fly stocks with visible markers on the chromosomes emphasized the potential of investigating genes in order to understand the development of organisms. By cloning the homeotic gene complexes, the concept of homeobox was revealed; a DNA binding domain for transcription factors that is conserved among both vertebrate and invertebrate organisms. This carried huge potential as it is one of the first clues of common patterns in organismal development.
Research in D. Melanogaster maternal mutants on the X chromosome revealed four groups of genes that specify the borders of the bodies of the flies. The axis determination shows significant differences in terms of biochemical composition, however, it has been observed that each gene group is dependent on cytoplasmic signals or a gradient of transcription factors which play a significant role in the expression of zygotic genes in a concentration-dependent manner (Wolf& Rockmann, 2008). These distinct genes that are important in developmental regulation have been observed to be homologous with human genes that are known to be related to development or disease progression (Tolwinski, 2017). Since research with human genes requires a lot more effort and extreme amounts of funding, D. Melanogaster remains an important tool for developmental biology research to justify human development.
Caenorhabditis elegans
Round worms, or nematodes are multicellular animals that either are males or hermaphrodites, meaning that they can fertilize on their own. The male species are generated less frequently than the females. In the 1960s, Sidney Brenner decided to use Caenorhabditis elegans as a bridge between unicellular and multicellular organisms due to its easy handling (Irion & Nüsslein-Volhard, 2022). Not only C. elegans C.elegans is small enough to be easily cultured in the laboratory, it has a low number of cells that can be utilized in lineage studies and genetic analysis. Since C. elegans C.elegans results in a high number of offsprings in every reproductive cycle, multiple alleles of the same mutant gene can be easily identified in a single experimental design. The mutant screens performed in C. elegans C.elegans in order to detect phenotypic changes remained to be successful indeed, the organism is the first animal in which Green Fluorescent Protein (GFP) was utilized as a gene expression marker.
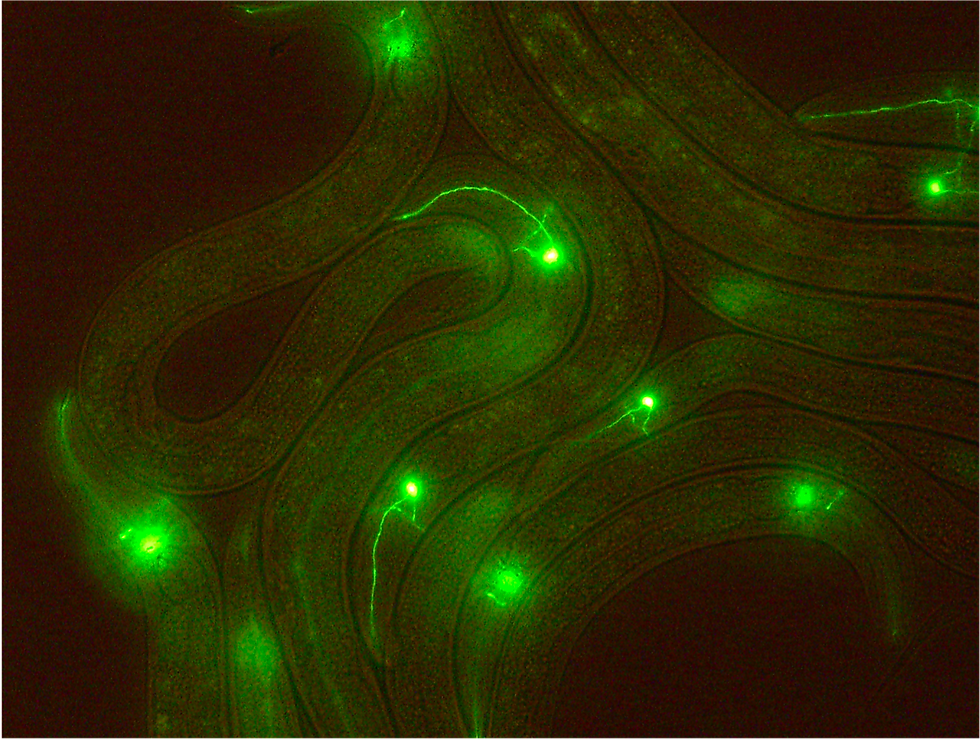
C.elegans is also the first animal whose genome is fully sequenced. In addition, the first mutagenic knock-down analysis of genes that encode small noncoding RNAs was first isolated from these roundworms (Irion & Nüsslein-Volhard, 2022). The proteomic similarities between the worm and other species explain why it can be such a powerful model organism that is utilized in developmental research.
Danio rerio
Aiming a similar approach as Brenner, George Streisinger chose zebrafish as a model organism to study the development of the nervous system in a vertebrate. Zebrafish was the perfect opportunity as it gives a high number of offsprings in a short period of time. Another advantage of Danio rerio is that the early embryos of these fish are transparent, making it optimal for visual analysis under the microscope. After the fertilization process, the organs can be visualized in 12 hours, so it is a potential model organism to acquire more knowledge about organs such as the eyes, the heart, the brain, etc. Unspecific lethal embryos (death happens in embryos prior to organogenesis) of the given species show pigmentation differences which can be seen through the microscope. This makes D. rerio a potential species to be analyzed in the laboratory. Since the embryos are transparent, analysis of fluorescent marker lines is fairly easy. Studies show significant genomic similarities between zebrafish and humans, making it an agent for pathologic research as well (Veldman &Lin, 2008).
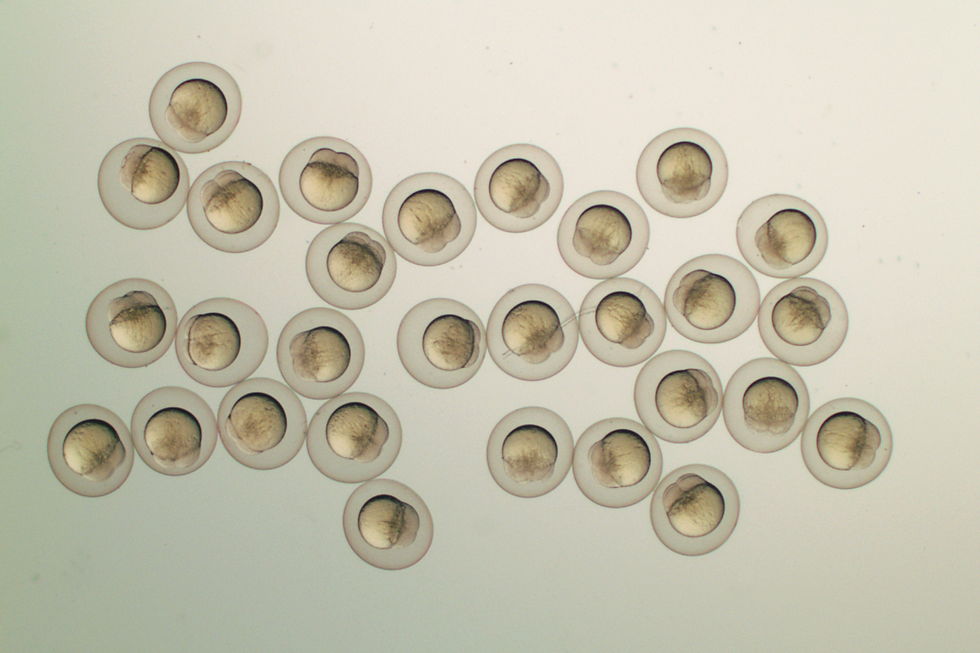
While the genome was being sequenced, multiple gene microarrays have been created in order to study gene expression at different levels. Application of chemical compounds to D. rerio contributes to research on animal physiology. By observing morphological changes in zebrafish embryos, chemical treatment options can be generated. Due to the reasons mentioned above, zebrafish serves as a significant model organism in developmental biology research.
Mus musculus
Mus musculus is one of the most commonly used model organisms in biological research. Although initial approaches were in the name of unraveling the secrets of cancer, the species was proven to be a significant model organism in various scientific systems. The species of mice that are used in the laboratory conditions is a hybrid whose genome is composed of a mixture of three subspecies of Mus musculus. These inbred strains were shown to acquire closer characteristics to humans than the other model organisms mentioned above. The reason for this phenomenon resides in the fact that male development in mice is determined by the Y chromosome, showing similarity to humans.
Another similarity is that X chromosome inactivation, a process where one of the X chromosomes is randomly picked and inactivated, is also intact in mouse models as expected from eutherian mammalians. After the development of embryonic stem cells, it became fairly easy for scientists to generate mutant mice lines by homologous recombination (Phifer-Rixey & Nachman, 2015). Today, it is even possible to generate knock-out lines of mice and perform reverse genetics procedures in order to understand the function of many genes that are yet unknown.
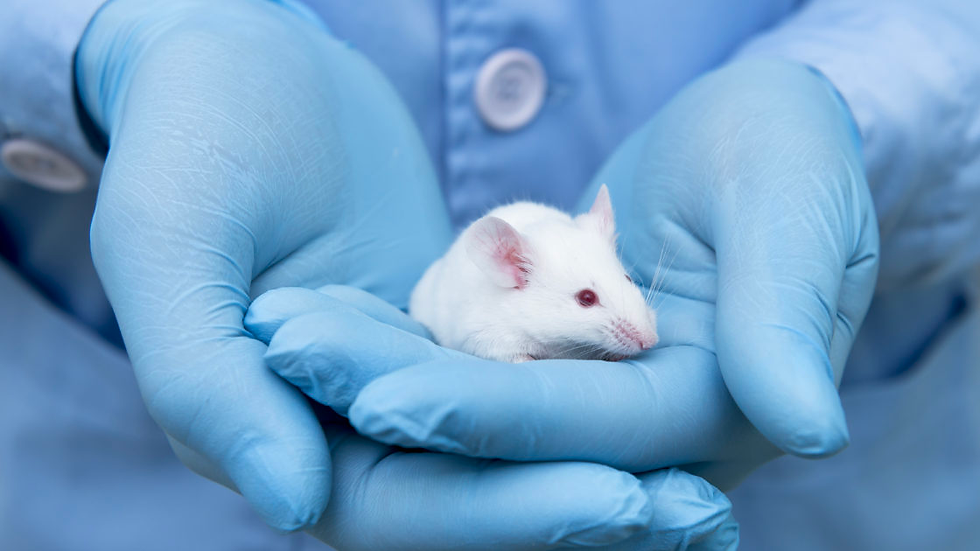
Arabidopsis thaliana
Due to its ease of use in genetic research, Arabidopsis thaliana emerges as a robust model system for investigating plant developmental processes. In the early approaches of molecular biology, Arabidopsis was considered to be advantageous over other plants due to its small genome size. It can also self-fertilize and can be transformed easily with bacteria in order to induce genetic manipulations. Stem cells of plants reside in special places within the organism called meristems. In their embryonic form, cells of A. thaliana give rise to a seedling composed of the first embryonic leaves (cotyledons), shoot, and root apical meristems (Woodward& Bartel, 2018). Studies on the shoot and root systems of Arabidopsis are shown to be essential for understanding the developmental processes of plant species.
In-vivo investigations of A. thaliana have demonstrated that the plant can thrive indoors under suitable light and watering conditions. This plant possesses all the observable attributes necessary for conducting phenotypic analyses, such as flowers, stems, and apical meristems. Moreover, certain gene families identified in A. thaliana have also been detected in other plant species, suggesting that research involving A. thaliana significantly contributes to comparative genomics and translational studies.
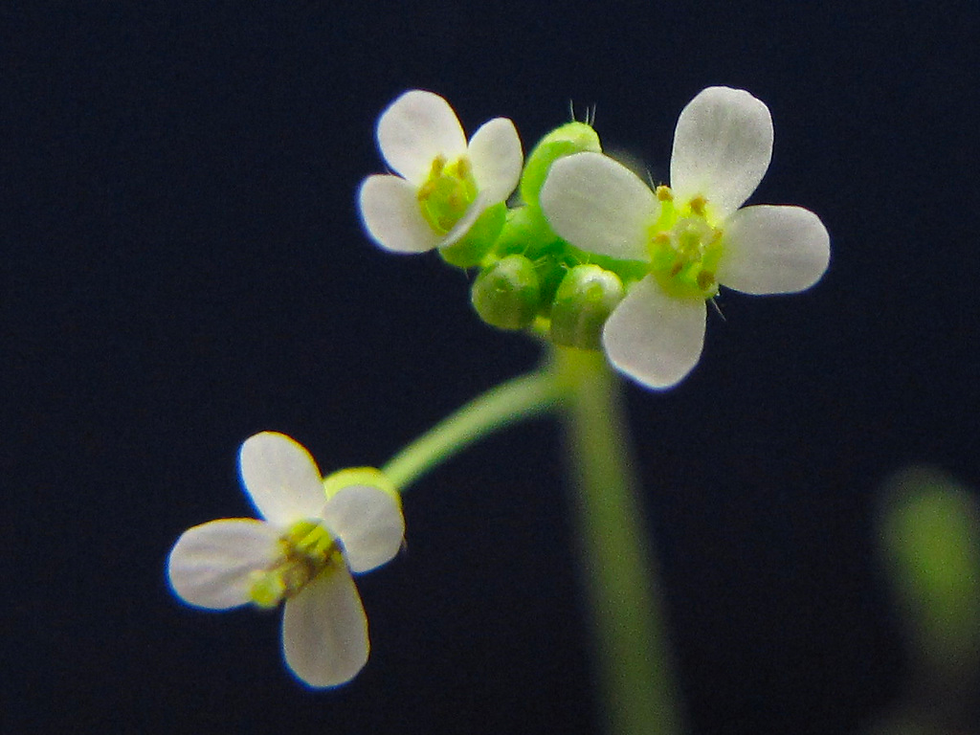
Xenopus laevis
Xenopus laevis, or African clawed frog has been utilized for centuries in modeling human diseases. The main reason for this is that the frog shows close relatedness to mammalians including humans, having lungs, and a three-chambered heart (Nenni et al., 2019). The embryos of the frog develop externally, meaning that the organismal development and performed manipulations can be observed fairly easily. Similar to D. rerio, X. laevis embryos also develop within a short period of time after fertilization which significantly enhances the pace of the ongoing research. The embryos are quite large and prosperous, leaving them eligible to perform experimental approaches such as microinjections and cell transplantation. Due to governing amphibian characteristics like transition from the aquatic to terrestrial environment, this model organism also acts as a potential model organism for evolutionary perspectives. Having an abundance of eggs, X. laevis is considered to be a cost-effective model organism that can be scientifically utilized.

The Future of Stem Cell Research
Stem cell research has a bright future and has the chance to change many industries, including medicine, regenerative medicine, disease modeling, and pharmaceutical development. By the generation of iPS cells, it may be possible to develop treatments specific for the patients by utilizing drug testing in regard to the genomic content of the individuals. Protocols are developed in order to understand if stem cell therapies have the potential to unravel the secrets behind unknown diseases of organisms including humans. Another potential use of stem cells is the generation of organoids in the laboratory environment, which would significantly reduce the number of donors required for organ transplants as well as the time needed for accurate treatment (Saini et al, 2009). If fully functional organs can be generated via reprogramming stem cells in the laboratory, the risks of the rejection of donor transplants by the immune system would be significantly reduced. As scientists continue to develop new approaches to stem cell research, ethical and legal issues will diminish, resulting in a healthy balance between scientific research and ethical considerations.
Conclusion
Developmental biology continues to unblur the intricacies of living beings by searching how the interplay of stem cells, morphogens, and niche interactions work together with what is gained from model organisms. This field holds promise for enhancing the universal understanding of development, regenerative medicine, and the fundamental mechanisms that shape life itself. Stem cells stand as the linchpin of developmental biology, playing a major role in the process of growth from a single cell to a complex multicellular organism. Morphogenesis is a significant player in the course of understanding how cells and tissues gain their functions, structures, and shapes. Model organisms, such as Drosophila melanogaster (fruit fly), Caenorhabditis elegans (roundworm), Danio rerio (zebrafish), Mus musculus (mouse), Arabidopsis thaliana (thale cress), and Xenopus laevis (African clawed frog), have been instrumental in advancing developmental biology research. A number of scientific fields, including medicine, regenerative medicine, disease modeling, and pharmaceutical development, stand to benefit from the promising future of stem cell research. Due to the above-mentioned reasons, stem cell research forms the foundation of developmental biology for developing a more profound understanding of how growth, regeneration, and aging are maintained throughout the life spans of different organisms.
Bibliographical References
Bagheri-Mohammadi, S. (2021). Stem cell-based therapy as a promising approach in Alzheimer's disease: current perspectives on novel treatment. Cell Tissue Bank 22, 339–353. https://doi.org/10.1007/s10561-020-09896-3
Blanpain, C., Horsley, V., & Fuchs, E. (2007). Epithelial stem cells: turning over new leaves. Cell, 128(3), 445–458. https://doi.org/10.1016/j.cell.2007.01.014
Bronner-Fraser M. (1994). Neural crest cell formation and migration in the developing embryo. FASEB journal : official publication of the Federation of American Societies for Experimental Biology, 8(10), 699–706. https://doi.org/10.1096/fasebj.8.10.8050668
Caplan A. I. (2015). Adult Mesenchymal Stem Cells: When, Where, and How. Stem cells international, 2015, 628767. https://doi.org/10.1155/2015/628767
Chagastelles, P. C., & Nardi, N. B. (2011). Biology of stem cells: an overview. Kidney international supplements, 1(3), 63–67. https://doi.org/10.1038/kisup.2011.15
Cyranoski D. (2018). How human embryonic stem cells sparked a revolution. Nature, 555(7697), 428–430. https://doi.org/10.1038/d41586-018-03268-4
Díez Villanueva, P., Sanz-Ruiz, R., Núñez García, A., Fernández Santos, M. E., Sánchez, P. L., & Fernández-Avilés, F. (2012). Functional multipotency of stem cells: what do we need from them in the heart? Stem cells international, 2012, 817364. https://doi.org/10.1155/2012/817364
Gilbert SF.(2000). Developmental Biology. 6th edition. Sunderland (MA): Sinauer Associates. Available from: https://www.ncbi.nlm.nih.gov/books/NBK9983/
Gurdon, J., Bourillot, PY.(2001). Morphogen gradient interpretation. Nature 413, 797–803 . https://doi.org/10.1038/35101500
Hawley, R. G., Ramezani, A., & Hawley, T. S. (2006). Hematopoietic stem cells. Methods in enzymology, 419, 149–179. https://doi.org/10.1016/S0076-6879(06)19007-2
He, S., Nakada, D., & Morrison, S. J. (2009). Mechanisms of stem cell self-renewal. Annual review of cell and developmental biology, 25, 377–406. https://doi.org/10.1146/annurev.cellbio.042308.113248
Irion, U., & Nüsslein-Volhard, C. (2022). Developmental genetics with model organisms. Proceedings of the National Academy of Sciences of the United States of America, 119(30), e2122148119. https://doi.org/10.1073/pnas.2122148119
National Research Council (US) and Institute of Medicine (US) Committee on the Biological and Biomedical Applications of Stem Cell Research. Stem Cells and the Future of Regenerative Medicine. Washington (DC): National Academies Press (US). (2002). CHAPTER THREE, Embryonic Stem Cells. Available from: https://www.ncbi.nlm.nih.gov/books/NBK223690/
Nenni, M. J., Fisher, M. E., James-Zorn, C., Pells, T. J., Ponferrada, V., Chu, S., Fortriede, J. D., Burns, K. A., Wang, Y., Lotay, V. S., Wang, D. Z., Segerdell, E., Chaturvedi, P., Karimi, K., Vize, P. D., & Zorn, A. M. (2019). Xenbase: Facilitating the Use of Xenopus to Model Human Disease. Frontiers in physiology, 10, 154. https://doi.org/10.3389/fphys.2019.00154
O'Donoghue, K., & Fisk, N. M. (2004). Fetal stem cells. Best practice & research. Clinical obstetrics & gynaecology, 18(6), 853–875. https://doi.org/10.1016/j.bpobgyn.2004.06.010
Pennings, S., Liu, K. J., & Qian, H. (2018). The Stem Cell Niche: Interactions between Stem Cells and Their Environment. Stem cells international, 2018, 4879379. https://doi.org/10.1155/2018/4879379
Phifer-Rixey, M., & Nachman, M. W. (2015). Insights into mammalian biology from the wild house mouse Mus musculus. eLife, 4, e05959. https://doi.org/10.7554/eLife.05959
Saini, R., Saini, S., & Sharma, S. (2009). Stem cell therapy: the eventual future. International journal of trichology, 1(2), 145–146. https://doi.org/10.4103/0974-7753.58562
Seifert, A. W., & Muneoka, K. (2018). The blastema and epimorphic regeneration in mammals. Developmental biology, 433(2), 190–199. https://doi.org/10.1016/j.ydbio.2017.08.007
Tolwinski N. S. (2017). Introduction: Drosophila-A Model System for Developmental Biology. Journal of developmental biology, 5(3), 9. https://doi.org/10.3390/jdb5030009
Torre, P., & Flores, A. I. (2020). Current Status and Future Prospects of Perinatal Stem Cells. Genes, 12(1), 6. https://doi.org/10.3390/genes12010006
Veldman, M., Lin, S. (2008). Zebrafish as a Developmental Model Organism for Pediatric Research. Pediatr Res 64, 470–476 . https://doi.org/10.1203/PDR.0b013e318186e609
Waas, B., & Maillard, I. (2017). Fetal hematopoietic stem cells are making waves. Stem cell investigation, 4, 25. https://doi.org/10.21037/sci.2017.03.06
Wolf, M. J., & Rockman, H. A. (2008). Drosophila melanogaster as a model system for genetics of postnatal cardiac function. Drug discovery today. Disease models, 5(3), 117–123. https://doi.org/10.1016/j.ddmod.2009.02.002
Woodward, A. W., & Bartel, B. (2018). Biology in Bloom: A Primer on the Arabidopsis thaliana Model System. Genetics, 208(4), 1337–1349. https://doi.org/10.1534/genetics.118.300755
Ye, L., Swingen, C., & Zhang, J. (2013). Induced pluripotent stem cells and their potential for basic and clinical sciences. Current cardiology reviews, 9(1), 63–72. https://doi.org/10.2174/157340313805076278
Zhao, X., & Moore, D. L. (2018). Neural stem cells: developmental mechanisms and disease modeling. Cell and tissue research, 371(1), 1–6. https://doi.org/10.1007/s00441-017-2738-1
Kaiser OTC benefits provide members with discounts on over-the-counter medications, vitamins, and health essentials, promoting better health management and cost-effective wellness solutions.
Obituaries near me help you find recent death notices, providing information about funeral services, memorials, and tributes for loved ones in your area.
is traveluro legit? Many users have had mixed experiences with the platform, so it's important to read reviews and verify deals before booking.