Stem Cell Biotechnology Series: Differentiation and Lineage Commitment
- Victor Cornily
- Aug 6, 2023
- 29 min read
Updated: Sep 11, 2024
Foreword
The field of stem cells is brimming with promise and intrigue. As the understanding of these remarkable foundational components of life continues to evolve, so does the capacity to harness their extraordinary potential. Stem cells have enchanted the minds of scientists, medical professionals, and curious individuals alike, presenting unparalleled opportunities to revolutionise healthcare and regenerative medicine. This series of articles aims to unravel the mysteries of stem cell differentiation, explore the mechanisms governing their self-renewal, and examine the factors that shape their destiny. Within these articles, readers may discover the immense potential of induced pluripotent stem cells (iPSCs) in reshaping personalised medicine while uncovering their awe-inspiring regenerative capabilities. Moreover, this series sheds light on the critical challenges and limitations inherently intertwined with this field, from ensuring the safety and efficacy of stem cell therapies through navigating the complex terrain of regulatory frameworks and public perception to debating ethical uncertainties.
This series is divided into eight articles, including:
3. Stem Cell Biotechnology Series: Differentiation and Lineage Commitment
Stem Cell Biotechnology Series: Differentiation and Lineage Commitment
What Does It Mean To Differentiate?
The focal point of this article delves into the complicated processes of differentiation and lineage commitment in stem cells. As previously discussed in this series, stem cells possess a unique capability to generate a variety of cell lineages, which is crucial for developing, maintaining, and restoring tissues and organs within the body. A complex interplay of interactions between cells, genetic programming, epigenetic control, and cues from the surrounding environment orchestrates this transformation.
Differentiation is the process of transforming stem cells into specialised cell types with distinguishable functions and characteristics. This process is fundamental for upkeeping tissues and organs in multicellular organisms. As stem cells divide and proliferate, they undergo changes in gene expression that lead to the activation of specific genes associated with a particular cell lineage. These alterations subsequently direct the cell's development towards a specific fate. Differentiation involves gradually restricting a cell's developmental potential, ushering it to acquire specific structural and functional attributes characteristic of its intended role within the body (Donnelly et al., 2018).
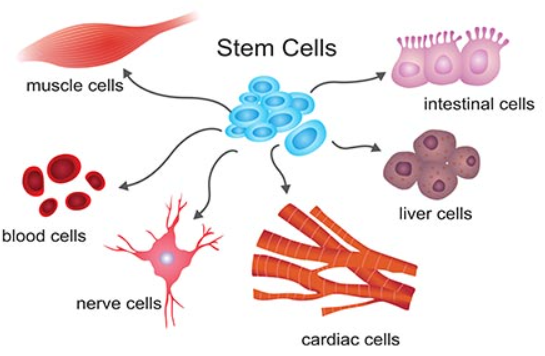
The Cell's Commitment
Lineage commitment represents a critical phase within differentiation, during which a stem cell dedicates itself to maturing into a distinct cell type or lineage. This commitment is carefully guided by complex molecular networks encompassing signalling pathways, transcription factors, and epigenetic modifications. These cues are crucial in guiding the cell onto an intended developmental trajectory. Once a stem cell embraces a specific lineage commitment, its range of developmental possibilities becomes progressively limited, ensuring the generation of specialised cell types essential for the organism's proper functioning (Loughran et al., 2020).
In simpler words, lineage commitment serves as the initial choice made by a stem cell to adopt a particular cellular identity. In contrast, differentiation embodies the actual transformative process through which the cell acquires the distinct traits and functions associated with that chosen cell type. Lineage commitment comes before differentiation and dictates the cell's path and fate. Differentiation is the observable consequence of that decisive commitment.
Differentiation During Embryonic Development
The cellular and molecular events that drive cell differentiation are highly orchestrated and intricately regulated during embryonic development. The transition from a zygote to a blastocyst involves distinct stages of specific cellular transformations and convoluted signalling interactions. Following fertilisation, the zygote undergoes rapid cleavage divisions, forming a solid mass of cells known as the morula. As development progresses and cell compaction occurs, the blastocyst is created, consisting of distinct cell populations. Within the blastocyst, the inner cell mass (ICM) contains pluripotent stem cells, which can give rise to all cell types in the body (Tyurin-Kuzmin et al., 2020). After the blastocyst is implanted into the uterus, trophoblast cells develop, leading to the formation of the chorion. Simultaneously, the inner cell mass differentiates into three germ layers, giving rise to various extra-embryonic organs such as the yolk sac, allantois, and amnion (Morgani et al., 2017).
A network of transcription factors tightly regulates the differentiation of pluripotent stem cells within the ICM (Peng & Abdel-Latif 2019). The most critical factors in early lineage commitment are Oct4, Sox2, and Nanog, with Oct4 being particularly crucial for maintaining pluripotency and preventing differentiation. These transcription factors interact with each other and the chromatin structure, orchestrating the activation or repression of specific gene expression patterns that steer cells toward particular lineages (Zakrzewski et al., 2019).

During embryonic development, the Wnt and Notch signalling pathways play vital roles in controlling cell patterning, ultimately determining cell fate and differentiation. These pathways also have significant regulatory roles in stem cell proliferation and differentiation in the mammalian intestine. In stem cells, Wnt and Notch signalling is active. However, their activity is polarised in progenitor cells, with absorptive progenitors showing low Wnt and high Notch activity, while secretory progenitors exhibit high Wnt and low Notch activity (Sancho et al., 2015). Research by Rodríguez-Colman et al. (2017) highlighted the importance of mitochondrial metabolism in maintaining colonic stem cells and their metabolic interactions with progenitor cells, emphasising the critical role of mitochondria in cell fate determination. When mitochondrial function is downregulated, stem cells differentiate into secretory lineages like goblet cells and Paneth cells, while absorptive enterocyte lineage differentiation remains unaffected. This mitochondrial regulation intersects with the FOXO signalling pathway. FOXO transcription factors play essential roles in intestinal homeostasis, and silencing FoxO1/3 alone is sufficient to drive secretory fate commitment (Ludikhuize et al., 2020). Furthermore, FOXO downregulation results in Notch inactivation, and conversely, inhibiting Notch reduces FOXO levels. The interaction between Notch and FOXO pathways significantly influences the inhibition of neural stem/progenitor cell differentiation, as observed in other biological systems (Hosaka et al., 2004). In addition, DRP1-dependent mitochondrial fission is crucial for embryonic and cellular differentiation in vivo and in vitro (Seo et al., 2018).
Polycomb Group Proteins
Polycomb group proteins (PcG) control gene expression during cell fate determination and embryonic development (Laugesen & Helin, 2014). These proteins form complexes categorised into two main functional groups: the Polycomb repressive complex 1 (PRC1) and the Polycomb repressive complex 2 (PRC2), both of which have active catalytic functions (Di Croce & Helin, 2013). PRC1 comprises several subunits, including members of the CBX family (Chromobox proteins). CBX proteins are essential for recognising specific chromatin marks and are key in guiding PRC1 to target genes. They contribute to the formation of repressive chromatin structures, leading to the silencing of lineage-specific genes, thereby preventing premature differentiation (Desai & Pethe, 2020). As stem cells progress towards lineage commitment, the composition of PRC1 complexes can change, and different CBX proteins may be incorporated to regulate the expression of specific lineage-associated genes (van Wijnen et al., 2021).
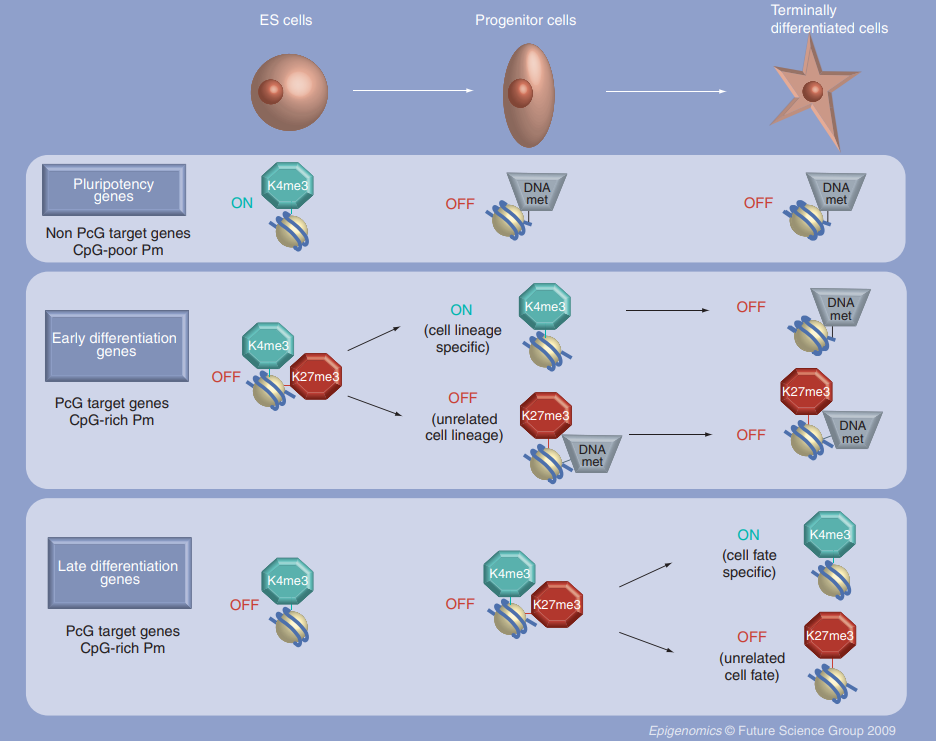
Among these proteins, CBX7, a stem cell-specific isoform of the CBX family, is highly expressed in stem cells to prevent premature differentiation by silencing lineage-specific genes (Jung et al., 2019). Depletion of CBX7 leads to differentiation and activation of lineage-specific markers, whereas its ectopic expression (an abnormal expression that does not typically happen) hinders the differentiation of embryonic stem cells (ESC) (O’Loghlen et al., 2012). As stem cells commit to specific lineages, CBX7 is replaced by CBX2, CBX4, and CBX8, which are expressed at increased levels during the differentiation of both ESCs and hematopoietic stem cells (HSCs). Overexpressing CBX2, CBX4, or CBX8 promotes the differentiation of pluripotent stem cells (Klauke et al., 2013). Mechanistically, the forced expression of CBX2 facilitates the compaction of nucleosome organisation, contributing to heterochromatin formation during ESCs differentiation (Tatavosian et al., 2019). CBX2 is essential for maintaining HSCs and progenitor self-renewal by repressing CDKN1A/p21 gene expression (van Wijnen et al., 2021). Moreover, CBX6, another abundantly expressed protein in ESCs, has been linked to rapid, spontaneous differentiation upon depletion in ESCs. CBX6 is required to maintain ESC identity (Santanach et al., 2017). Additionally, HP1-related CBX proteins (CBX1, CBX3, CBX5) fulfil distinct roles in stem cell identity and differentiation, with CBX1 and CBX3 involved in pluripotency maintenance and differentiation regulation (Mattout et al., 2015). CBX5 accelerates endothelial stem/progenitor cell differentiation into distinct endothelial cell types (Maeng et al., 2015).
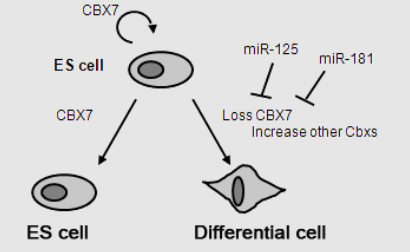
PRC2 comprises three core subunits. Together, these subunits work to catalyse the methylation of histone H3, forming repressive chromatin domains. This mark helps silence or turn off certain genes, which is crucial for keeping stem cells undifferentiated. As cells commit to becoming different types, the PRC2 complex can change its activity, leading to different gene expression patterns. PRC2 interacts with other proteins and factors that control gene activity, ensuring the right genes are turned on or off at the right time. While PRC2 is mainly known for silencing genes, it can also activate specific genes depending on the context (Laugesen et al., 2019). The balance between PRC1 and PRC2, along with the involvement of different CBX proteins in PRC1, helps to control gene expression during stem cell development, carefully guiding cells to become specific cell types (van Wijnen et al., 2021).
Metabolism as a Key Player
Significant changes occur in how cells handle metabolic processes during stem cell differentiation. Metabolic enzymes such as NADH and acetyl coenzyme A (acetyl-CoA) are necessary for the regulation of stem cell properties. Metabolic processes act as regulatory mechanisms that determine how stem cells participate in tissue formation and maintain homeostasis (Tyurin-Kuzmin et al., 2020). At first, scientists believed that the unique metabolic features of stem cells were simply a result of their environmental conditions. For example, early-stage embryos, like the zygote, morula, and early blastocyst, primarily synthesise ATP by metabolising lactate, pyruvate, amino acids, and fatty acids from their surroundings (Ryall et al., 2015). As the embryo develops and oxygen availability decreases, the cells switch to anaerobic metabolism (Houghton et al., 1996). With the development of the placenta, tissues receive sufficient oxygen, and cells increasingly rely on oxidative phosphorylation for ATP production during differentiation. In adult organisms, postnatal stem cells reside in specialised microenvironments called niches, where oxygen and nutrient availability can vary. Many are characterised by limited oxygen and nutrients, leading to a significant reliance on the glycolytic pathway for energy metabolism in postnatal stem cells (Novoseletskaya et al., 2019).
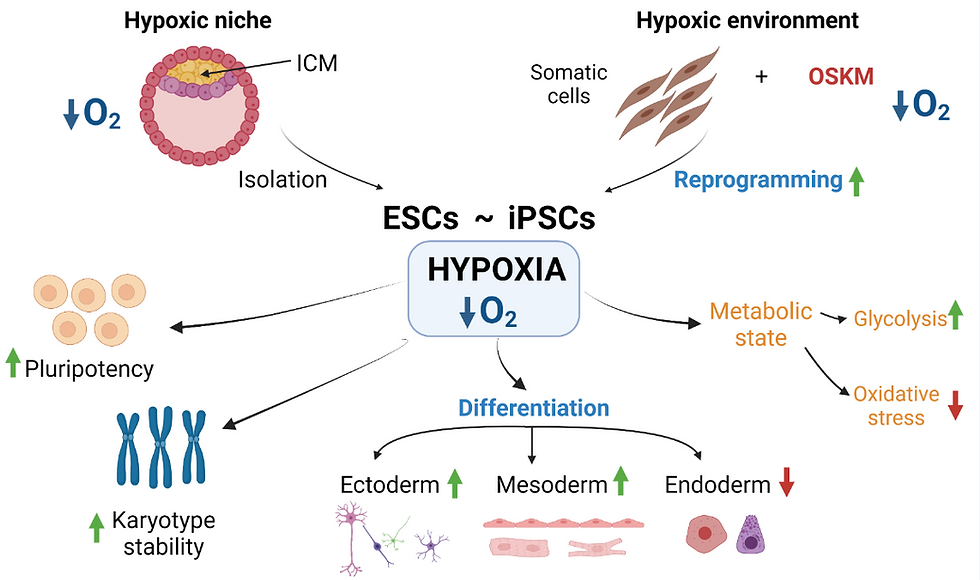
As aforementioned, during cell differentiation, there is a shift in the cellular energy production strategy. Initially, cells predominantly rely on glycolysis, a metabolic process that converts sugar into energy. As they differentiate, cells switch to oxidative phosphorylation, which occurs within the mitochondria. In oxidative phosphorylation, pyruvate, a product of glycolysis, undergoes conversion into acetyl-CoA, also within the mitochondria. This metabolic pathway generates NADH and FADH2 molecules, which serve as energy carriers. The energy carried by these molecules contributes to establishing an electrochemical gradient across the inner mitochondrial membrane, resembling a storage battery for energy. In the final step of this process, oxygen acts as the terminal electron acceptor in the respiratory chain, where it accepts electrons from the energy-carrying molecules. The efficient functioning of this entire system is crucial for cells to sustain their proper function and energy production (Tyurin-Kuzmin et al., 2020).
A multitude of metabolites exert significant influence over cell fate determination. Alterations in their concentrations and ratios serve as metabolic cues that impact the activity of enzymes and transcription factors (Ryall et al., 2015). For instance, low glucose levels and high lactate-to-pyruvate ratios can trigger the activation of hypoxia-inducible factor 1 alpha (HIF-1α), a master regulator of cellular responses to low oxygen conditions. HIF-1α governs the expression of genes involved in glycolysis and cellular adaptation to hypoxic environments, influencing the decisions cells make regarding their fate. When oxygen levels are low (hypoxia), HIF-1α stabilises and activates specific genes that help cells adapt to low oxygen conditions. In stem cell differentiation, HIF-1α plays a role in guiding cells towards specific lineages. It can affect the balance between stem cell self-renewal and differentiation by regulating gene expression in cell fate determination. When HIF-1α is activated, it can influence the decision of stem cells to either maintain their stemness or differentiate into specialised cell types depending on the specific microenvironmental conditions (Zhang et al., 2022).
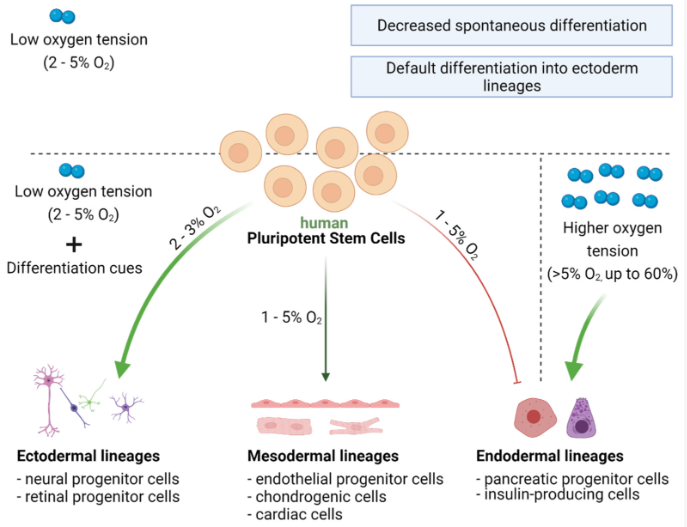
Altering the balance between metabolites can induce cells to acquire specific phenotypes, including stem cell characteristics. For instance, during capillary angiogenesis, endothelial cells differentiate into two distinct types: apical cells responsible for capillary growth and wall cells forming the capillary walls. Their dominant metabolic pathways control this differentiation. Apical cells rely on glycolysis as their primary energy source, while capillary wall cells utilise oxidative phosphorylation. Intriguingly, when glycolysis is experimentally activated in capillary wall cells, they rapidly transdifferentiate into new apical cells, forming capillary branching points (Rivera & Bergers, 2014). Cell differentiation and transdifferentiation are constantly occurring in the body, and they are influenced decisively by the availability of energy substrates, ATP production rate, and the resulting metabolites (Tyurin-Kuzmin et al., 2020).
One way metabolites dictate cell differentiation is when certain molecules' concentration in the cell changes, which activates specific transcription factors that control the activity of genes. For example, when there is an increase in fatty acids in the cell, a protein called SREBP decreases its activity, which affects the differentiation of specific cells (Kim et al., 2002). Notably, some enzymes that help break down sugars can also directly affect the activity of genes. For instance, an enzyme called GAPDH helps with sugar breakdown, but it also controls when certain genes are turned on or off during the S-phase of the cell cycle (Zheng et al., 2003). Many enzymes that help with gene activity require specific molecules like acetyl-CoA, ATP, and NADH to work correctly. These molecules act like messengers, helping to coordinate gene activity with the cell's metabolism (Tyurin-Kuzmin et al., 2020).
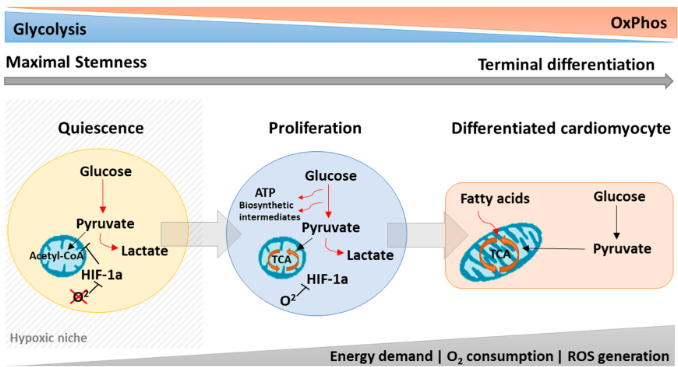
The influence of metabolites on cell differentiation is most widely studied through the regulation of epigenetic modifications involving histones and DNA. A crucial epigenetic change is histone acetylation, which is closely related to the intensity of glycolysis. During glycolysis, pyruvic acid changes into acetyl-CoA, which acts as a donor of acetyl groups for histone acetylases (Ryall et al., 2015).
Many tissues and organs in the body contain their unique group of stem cells, such as hematopoietic, neural, stomach, intestine, and muscle stem cells (MuSCs). Unlike embryonic stem cells, most adult stem cells (ASCs) are tissue-specific and can undergo asymmetric division, producing a committed daughter cell and a stem cell capable of self-renewal (Goulas et al., 2012; Rocheteau et al., 2012). Interestingly, tissue-specific stem cells exhibit varying turnover rates, with some constantly dividing while others remain in a quiescent state, only becoming active in response to tissue damage. Additionally, the local environment or niche surrounding ASCs can differ regarding substrate availability, oxygen levels, pH, and other factors. Despite their importance, the metabolic status of these tissue-specific stem cells and how the local metabolic conditions may regulate their biology remain largely unknown. The metabolic state of quiescent stem cell populations like adult hematopoietic stem cells and MuSCs is particularly intriguing. While increased reliance on glycolysis seems common during phases of cell proliferation in most ASCs, the metabolic status of quiescent cells is an area of growing interest and exploration (Ryall et al., 2015).
Two main categories of pluripotent stem cells (PSCs) have been obtained from embryonic sources: the 'naive' or 'ground-state' and the 'primed' state, which has distinct metabolic features. Both types of PSCs show reduced mitochondrial content and lower inner mitochondrial membrane potential compared to differentiated cells (Prigione & Adjaye, 2011). Human and murine-primed PSCs exhibit a different metabolic profile, resembling early post-implantation/epiblast stage cells (Nichols & Smith, 2009). Unlike naive PSCs, which largely utilise oxidative phosphorylation for energy generation, they predominantly rely on glycolysis for ATP production (Varum et al., 2011). In other words, primed PSCs rely on glycolysis, whilst naive PSCs make use of oxidative phosphorylation (Ryall et al., 2015).

The metabolic distinctions between naive and primed PSCs have significant functional implications. The reliance on glycolysis in primed PSCs may reflect their unique developmental state and cellular functions. Glycolysis is a less efficient energy production pathway than oxidative phosphorylation; however, it allows primed PSCs to rapidly generate ATP, which may be advantageous during their proliferation phase and in response to environmental signals. Contrarily, naive PSCs' reliance on oxidative phosphorylation suggests a more energy-efficient and quiescent state, which may be related to their self-renewal and pluripotency maintenance characteristics (Ryall, 2015).
Epigenetics
Epigenetics encompasses a complex regulatory framework that modulates gene expression through biochemical modifications to DNA and associated proteins. This regulatory system functions analogously to a multifaceted control apparatus for DNA. Genes are akin to an extensive library of genetic information represented by DNA sequences. At the same time, epigenetic processes resemble strategic markers or annotations similar to bookmarks, selectively designating which portions of this metaphoric library should be transcribed or remain transcriptionally silent. This orchestration can be influenced by a spectrum of external factors encompassing environmental cues, lifestyle factors, and experiential inputs (Li, 2021). In the context of guiding stem cells towards their fate, specific genes must be activated or suppressed to enable the cell to execute its specialised functions. Here, epigenetic modifications function as switches that regulate the accessibility of genes to the cellular machinery responsible for executing gene expression (Urbán, 2021).
Histones are also modified through a process called deacetylation, which involves enzymes known as histone deacetylases. One specific group of these enzymes, called sirtuins or class III histone deacetylases, relies on NAD+ levels in the cell for their activity. Sirtuins act as cellular sensors and respond to changes in the NAD+/NADH potential, which occurs when cells switch between glycolysis and oxidative phosphorylation. When glycolysis is more active, the NAD in the cytoplasm becomes more reduced. As cells transition to oxidative phosphorylation, the increased NAD+ fraction is sensed by sirtuins, leading to the deacetylation of histones and other proteins (Cantó et al., 2015).
The balance between histone acetylation and deacetylation is crucial for controlling gene expression during stem cell differentiation. This delicate balance impacts how stem cells develop into different cell types. Some acetylation patterns can maintain stem cell pluripotency, while some deacetylation patterns can drive stem cell specialisation. Precise regulation of these epigenetic modifications is vital for guiding stem cell fate and function as they grow and maintain tissues. The ratio of acetylated to deacetylated histones can vary depending on the particular cell conditions, developmental stage, and environmental signals. This dynamic and reversible process enables cells to swiftly respond to their surroundings and adjust their gene activity accordingly (Sellmer et al., 2018; Shahbazian & Grunstein, 2007).
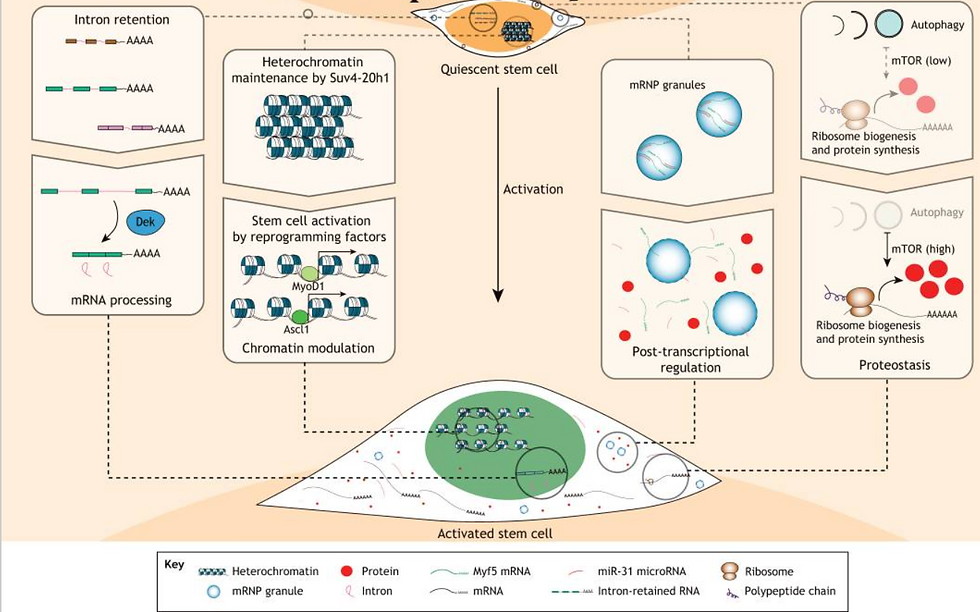
The activity of microRNAs (miRNAs) plays a crucial role in modulating the expression of cell cycle-associated genes. MiRNAs, a class of small non-coding RNAs, are implicated in finely tuning various biological processes and are widely expressed across plants, animals, and viruses, indicating their evolutionary significance (Wang & Blelloch, 2011). In humans, 1881 miRNAs have been confidently identified in the miRBase database (v.21), and they are thought to regulate the expression of more than 60% of all protein-coding genes (Kozomara & Griffiths-Jones, 2014). Research has shown that miRNAs play essential functional roles in cell proliferation, apoptosis, and differentiation mechanisms. Moreover, alterations in miRNA expression have been linked to various human diseases, including cancer and cardiovascular disease (Ghanbari et al., 2015). In embryonic stem cells, a specific set of miRNAs is expressed, with some being abundantly present at levels of 60,000 or more copies per cell. These miRNAs are critical in regulating the self-renewal and differentiation processes of ESCs, contributing to their pluripotency and potential to give rise to various cell types in the body (Mens & Ghanbari, 2018).
Circular RNAs (circRNAs) are a distinct form of genetic material found in cells, including stem cells. Their unique circular structure grants them stability and protection from breakdown. They are highly present in different cell types, including stem cells, and have essential roles in controlling gene expression and cellular activities. While scientists continue to investigate how circRNAs influence stem cell differentiation, the emerging evidence underscores their importance in shaping the fate of stem cells (Lin et al., 2021).
The Extracellular Matrix
In addition to the inherent characteristics within a cell, external signals from its surrounding microenvironment play significant roles in determining the cell's fate during the differentiation process. The extracellular matrix (ECM) is a dynamic and complex network of proteins and proteoglycans that surrounds cells. From the moment of oocyte fertilisation, the ECM undergoes constant renewal. In mammals, the oocyte is surrounded by a thick layer of ECM called the zona pellucida. New proteins are secreted upon fertilisation, which is essential for subsequent embryo implantation and development. Knocking out genes encoding ECM proteins results in embryonic death or severe developmental disorders (Novoseletskaya et al., 2019).
In the postnatal phase of life, ECM still supports cell activity, including stem cells. The ECM is a three-dimensional network formed by fibrillar proteins such as collagen, elastin, tenascins, and fibronectin, embedded in a three-dimensional porous gel formed by nonfibrillar collagen and other proteoglycans. Depending on the tissue type, the ratio of fibrillar proteins to gel, fibrillar network density, pore size, and distribution in the proteoglycan gel can vary significantly (Yi et al., 2017).
In the human body, the renewal of various tissues relies on the proliferation and differentiation of specialised tissue-specific stem cells residing in distinct microenvironments called stem cell niches. Schofield first proposed the concept of the stem cell niche in 1978, although his work concentrated on HSCs (Schofield, 1978). As research progressed, the idea was extended to other types, such as neural stem cells, mesenchymal stem cells, and epithelial stem cells, among others (Spit et al., 2018). Modern understanding of the stem cell niche suggests that it is not a static environment but a dynamic one (Donnelly et al., 2018).
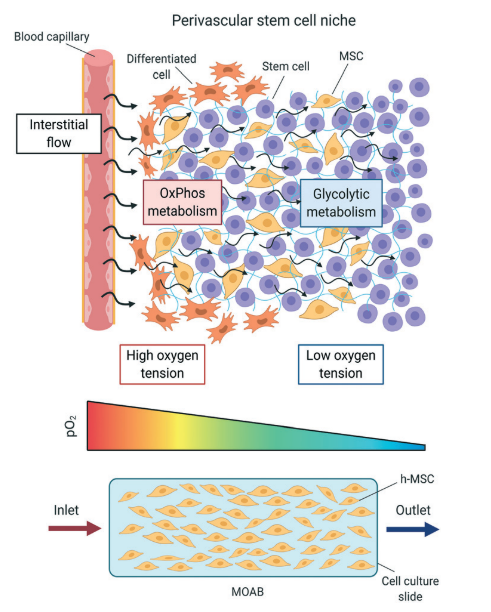
The stem cell niche can activate stem cells in response to various external signals. These signals may come from neighbouring cells or the ECM itself. When specific cues are received, stem cells are triggered to undergo proliferation and differentiation, leading to the generation of specialised cell types required for tissue repair and regeneration. Besides, the niche is not isolated from other cellular activities within the tissue. The ECM is continuously being remodelled and modified by cells that synthesise and degrade its components. This remodelling process can impact stem cell behaviour and responses and influence overall tissue homeostasis (Novoseletskaya et al., 2019).
Importantly, stem cell lineage commitment is not solely determined by intrinsic factors within the stem cell itself (Ahmed & ffrench-Constant, 2016). In the niche, stem cells interact with neighbouring cells, such as other stem cells, differentiated cells, and supporting cells like stromal cells or immune cells. These interactions involve the exchange of signalling molecules, cell-surface ligands, and extracellular vesicles. Such communication enables the niche to regulate stem cell fate decisions by providing instructive signals or physical cues. These cell-cell interactions are highly dynamic and context-dependent. Paracrine signalling, where neighbouring cells secrete signalling molecules, can activate or inhibit specific signalling pathways in stem cells. For instance, ligands from adjacent cells can bind to receptors on the stem cell surface, activating intracellular signalling cascades that dictate the cell's response (Liu et al., 2016).
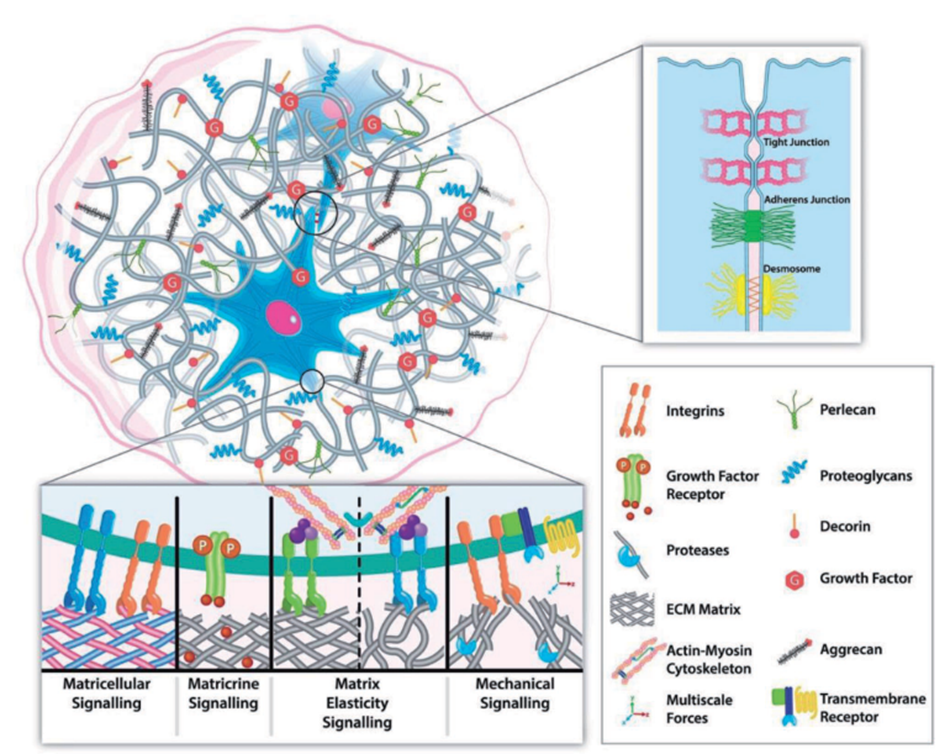
The ECM communicates with stem cells through cell surface receptors called integrins, sensitive to specific ECM components. Activation of integrins triggers intracellular signalling cascades that regulate gene expression, determining the fate of stem cells. Various ECM proteins, such as fibronectin, laminin, and collagens, bind to integrins on the cell surface, initiating downstream signalling events (Kechagia et al., 2019). For instance, ECM-bound growth factors, such as transforming growth factor-beta (TGF-β) and fibroblast growth factors, are sequestered and presented to the stem cells, influencing their differentiation processes. ECM-bound TGF-β can activate the Smad signalling pathway, upregulating lineage-specific transcription factors. Additionally, ECM proteins can serve as reservoirs for growth factors, protecting them from degradation and regulating their release to control stem cell behaviour (Yao et al., 2019).
The physical properties of the ECM, such as its stiffness and composition, also impact stem cell differentiation. Stiff ECM substrates promote osteogenic differentiation, while soft substrates favour neural differentiation. Furthermore, the spatial organisation of the ECM influences cell polarity and tissue architecture, which are essential for proper lineage commitment during development (Jiang et al., 2022). Remarkably, the ECM is not only a passive scaffold but also actively responds to cellular cues and modifies its composition and organisation in response to changes in the microenvironment. For example, matrix metalloproteinases secreted by stem cells and other cells in the niche can degrade ECM proteins, releasing growth factors and exposing cryptic sites, thereby influencing stem cell differentiation (Wu et al., 2022).
In addition to the mentioned mechanisms, the ECM's role in stem cell differentiation is further influenced by the presence of specific ECM-binding proteins and proteoglycans. These proteins can interact with stem cell surface receptors, activating intracellular signalling pathways and influencing cell behaviour. For instance, certain ECM-bound proteins, like N-cadherin and vitronectin, promote cell adhesion and cell-cell interactions, creating microenvironments that favour stem cell maintenance or differentiation (Chen et al., 2011).
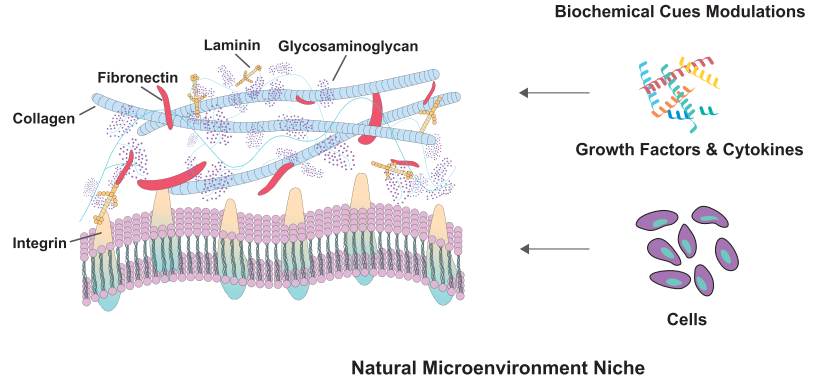
Moreover, the spatial organisation and topography of the ECM also play essential roles in stem cell fate determination. Cells sense the physical cues provided by the ECM's architecture, such as the presence of nanotopographical features, and respond by altering their gene expression profiles. These responses can direct stem cells towards specific lineages or maintain them undifferentiated. The ECM's topographical features can mimic the natural tissue environment and guide stem cell differentiation towards tissue-specific cell types (Thomas et al., 2018).
Additionally, the ECM's interaction with various signalling pathways, including Wnt and Notch, further influences stem cell fate decisions. ECM components can modulate the activity of these pathways and, in consequence, regulate the expression of genes that control cell differentiation. Furthermore, the ECM's ability to sequester and release growth factors, cytokines, and other signalling molecules also contributes to the finely tuned regulation of stem cell behaviour and lineage commitment (Ludikhuize et al., 2020).
Apart from its influence on cell migration and division symmetry, the ECM is also crucial for distributing mechanical forces within the tissue microenvironment. Stem cells can detect mechanical cues and changes in ECM elasticity, which elicits intracellular signalling events (Gilbert et al., 2010). The most extensively studied mechanism involves integrin-mediated focal contact assembly, leading to the reorganisation of the cytoskeleton and activation of Rho-GTPase. This process can directly impact gene transcription by engaging nuclear membrane proteins like nesprins, which subsequently signal to lamin A, modulating gene expression. Increased ECM stiffness, resulting from enhanced lamin A expression, triggers osteogenic differentiation in mesenchymal stem cells (Swift et al., 2013). Likewise, Rho-GTPase can activate the Hippo signalling pathway, involving YAP and TAZ proteins, which mediate stem cell differentiation in response to ECM mechanical cues and promote the proliferation of intestinal epithelial stem cells. Mamidi et al., 2018 have elucidated the role of ECM interactions with αV integrin/F-actin/YAP1/Notch signalling axis components in determining the differentiation fate of pancreatic progenitor cells.
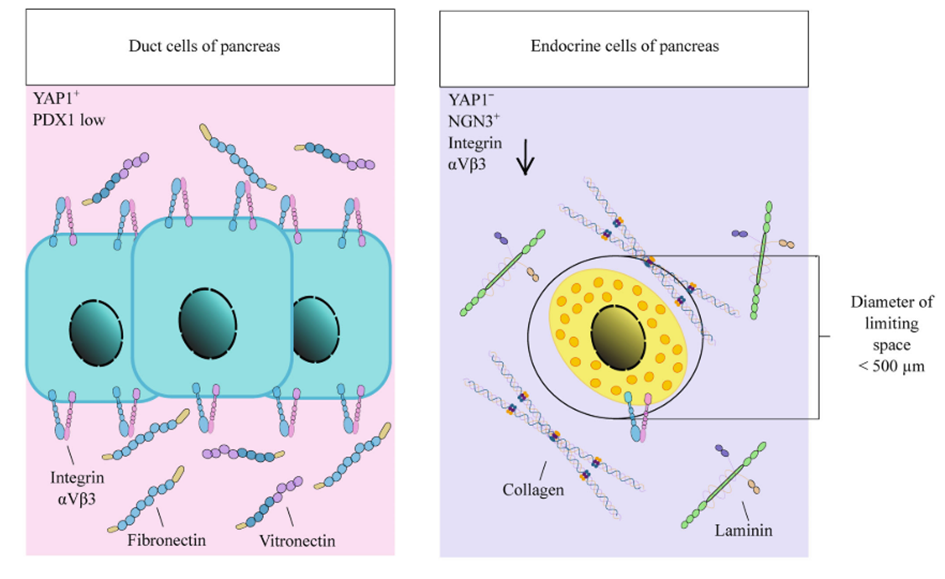
As already mentioned, the meticulous composition and structural organisation of the extracellular matrix wield considerable influence over the destiny and differentiation of stem cells. Subtle variations in the relative proportions of distinct protein isoforms within the ECM can profoundly affect stem cell behaviour. For instance, shifts in the balance between laminin 332 and laminin 511 within the epidermal stem cell niche trigger the activation of specific signalling pathways, culminating in promoting cell differentiation (Ahmed & ffrench-Constant, 2016). During wound healing and hair follicle development, laminin 332 undergoes specialised proteolytic cleavage, which is essential for facilitating keratinocyte migration (Sugawara et al., 2008). Similarly, the ECM composition within the eye limbus's crypts governs mitotic spindles' orientation in limbal stem cells and their subsequent migration and differentiation. Also, the ECM can effectively induce the differentiation of hair follicle stem cells into corneal epithelial cells (Nowell & Radtke, 2017).
An essential aspect of the ECM's influence on stem cell differentiation is the presentation and function of extracellular vesicles (EVs). Anchored within the ECM by integrins, EVs are pivotal in mediating the ECM's effects on stem cells. By selectively binding to specific recipient cells through interactions with surface receptors, EVs stimulate intracellular signalling, activating pathways that govern cell differentiation (Novoseletskaya et al., 2019). These EVs can generate local gradients of Wnt as they diffuse throughout the ECM and facilitate the transfer of Wnt between cells (Shapiro et al., 2015).
It is noteworthy to describe the exchange of EVs between stem cells and their neighbouring cells. Such EV-mediated communication enables the transfer of regulatory and messenger RNAs, opening the possibility of cell reprogramming and influencing stem cell fate. In the context of tumour stem cells, EVs serve as instrumental agents in preparing the stromal microenvironment and establishing distant metastatic niches. While the phenomenon of "niche translocation" remains somewhat enigmatic, it is increasingly evident that the ECM plays a central role in modulating EV production and release (Novoseletskaya et al., 2019). Combining specific ECM for osteoblasts or adipocytes with EVs isolated from these cells enhances the expression of marker genes in mesenchymal stem cells more efficiently than using ECM or EVs separately. This finding implies that ECM and EVs work synergistically to regulate stem cell fate and differentiation (Narayanan et al., 2018).
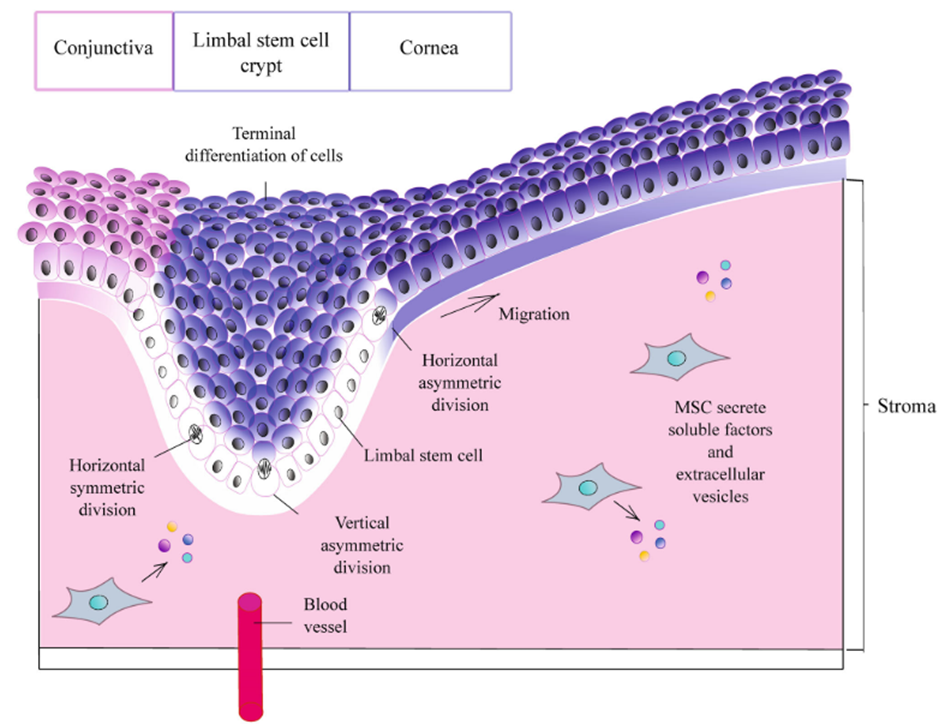
To put it succinctly, the extracellular matrix's elaborate interplay with stem cells, encompassing interactions involving integrins, growth factors, physical properties, and topographical cues, is of paramount importance in orchestrating and guiding these cells towards their specific fate and developmental pathways.
Conclusions
Stem cell lineage commitment is a highly orchestrated and complex process tightly regulated by a myriad of cellular and environmental factors. The cellular events during embryonic development, involving specific cellular transformations and intricate signalling interactions, give rise to distinct cell populations with diverse differentiation potentials. Metabolic processes and the cellular microenvironment are critical players in stem cell fate decisions. The metabolic status of stem cells, whether quiescent or actively dividing, significantly influences their differentiation potential. The extracellular matrix and cell-cell interactions within the niche also guide stem cells towards particular lineages. Signals from neighbouring cells and external environmental cues also impact stem cell fate choices, further adding to the complexity of lineage commitment. Moreover, epigenetic features, particularly microRNAs, fine-tune gene expression during cell cycle regulation and differentiation. A comprehensive understanding of these interconnected mechanisms is crucial for advancing stem cell biotechnology and regenerative medicine, paving the way for transformative therapeutic strategies to improve human health and address various diseases and injuries.
Bibliographical References
Ahmed, M., & ffrench-Constant, C. (2016). Extracellular Matrix Regulation of Stem Cell Behavior. Current Stem Cell Reports, 2(3), 197–206. https://doi.org/10.1007/s40778-016-0056-2
Cantó, C., Menzies, K. J., & Auwerx, J. (2015). NAD+ Metabolism and the Control of Energy Homeostasis: A Balancing Act between Mitochondria and the Nucleus. Cell Metabolism, 22(1), 31–53. https://doi.org/10.1016/j.cmet.2015.05.023
Chen, G., Gulbranson, D. R., Hou, Z., Bolin, J. M., Ruotti, V., Probasco, M. D., Smuga-otto, K., Howden, S. E., Nicole, R., Propson, N. E., Wagner, R., Lee, G. O., Teng, J. M. C., & Thomson, J. A. (2011). Chemically defined conditions for human iPS cell derivation and culture. Nat Methods, 8(5), 424–429. https://doi.org/10.1038/nmeth.1593.Chemically
Desai, D., & Pethe, P. (2020). Polycomb repressive complex 1: Regulators of neurogenesis from embryonic to adult stage. Journal of Cellular Physiology, 235(5), 4031–4045. https://doi.org/10.1002/jcp.29299
Di Croce, L., & Helin, K. (2013). Transcriptional regulation by Polycomb group proteins. Nature Structural and Molecular Biology, 20(10), 1147–1155. https://doi.org/10.1038/nsmb.2669
Donnelly, H., Salmeron-Sanchez, M., & Dalby, M. J. (2018). Designing stem cell niches for differentiation and self-renewal. Journal of the Royal Society Interface, 15(145). https://doi.org/10.1098/rsif.2018.0388
Donnelly, H., Salmeron-Sanchez, M., & Dalby, M. J. (2018). Designing stem cell niches for differentiation and self-renewal. Journal of the Royal Society Interface, 15(145). https://doi.org/10.1098/rsif.2018.0388
Ghanbari, M., Franco, O. H., De Looper, H. W. J., Hofman, A., Erkeland, S. J., & Dehghan, A. (2015). Genetic Variations in MicroRNA-Binding Sites Affect MicroRNA-Mediated Regulation of Several Genes Associated with Cardio-metabolic Phenotypes. Circulation: Cardiovascular Genetics, 8(3), 473–486. https://doi.org/10.1161/CIRCGENETICS.114.000968
Gilbert, P., Havenstrite, K., Magnusson, K., & Sacco, A. (2010). Substrate elasticity regulates skeletal muscle stem cell self- renewal in culture. Science, 329(5995), 1078–1081. https://doi.org/10.1126/science.1191035.Substrate
Goulas, S., Conder, R., & Knoblich, J. A. (2012). The par complex and integrins direct asymmetric cell division in adult intestinal stem cells. Cell Stem Cell, 11(4), 529–540. https://doi.org/10.1016/j.stem.2012.06.017
Hosaka, T., Biggs, W. H., Tieu, D., Boyer, A. D., Varki, N. M., Cavenee, W. K., & Arden, K. C. (2004). Disruption of forkhead transcription factor (FOXO) family members in mice reveals their functional diversification. Proceedings of the National Academy of Sciences of the United States of America, 101(9), 2975–2980. https://doi.org/10.1073/pnas.0400093101
Houghton, F. D., Thompson, J. G., Kennedy, C. J., & Leese, H. J. (1996). Oxygen consumption and energy metabolism of the early mouse embryo. Molecular Reproduction and Development, 44(4), 476–485. https://doi.org/10.1002/(SICI)1098-2795(199608)44:4<476::AID-MRD7>3.0.CO;2-I
Jiang, Y., Zhang, H., Wang, J., Liu, Y., Luo, T., & Hua, H. (2022). Targeting extracellular matrix stiffness and mechanotransducers to improve cancer therapy. Journal of Hematology and Oncology, 15(1), 1–15. https://doi.org/10.1186/s13045-022-01252-0
Jung, J., Buisman, S. C., Weersing, E., Dethmers-Ausema, A., Zwart, E., Schepers, H., Dekker, M. R., Lazare, S. S., Hammerl, F., Skokova, Y., Kooistra, S. M., Klauke, K., Poot, R. A., Bystrykh, L. V., & de Haan, G. (2019). CBX7 Induces Self-Renewal of Human Normal and Malignant Hematopoietic Stem and Progenitor Cells by Canonical and Non-canonical Interactions. Cell Reports, 26(7), 1906-1918.e8. https://doi.org/10.1016/j.celrep.2019.01.050
Kechagia, J. Z., Ivaska, J., & Roca-Cusachs, P. (2019). Integrins as biomechanical sensors of the microenvironment. Nature Reviews Molecular Cell Biology, 20(8), 457–473. https://doi.org/10.1038/s41580-019-0134-2
Kim, H., Miyazaki, M., Man, W. C., & Ntambi, J. M. (2002). Sterol Regulatory Element-Binding Proteins (SREBPs) as Regulators of Lipid Metabolism. New York Academy of Sciences, 967, 34–42.
Klauke, K., Radulović, V., Broekhuis, M., Weersing, E., Zwart, E., Olthof, S., Ritsema, M., Bruggeman, S., Wu, X., Helin, K., Bystrykh, L., & De Haan, G. (2013). Polycomb Cbx family members mediate the balance between haematopoietic stem cell self-renewal and differentiation. Nature Cell Biology, 15(4), 353–362. https://doi.org/10.1038/ncb2701
Kozomara, A., & Griffiths-Jones, S. (2014). MiRBase: Annotating high confidence microRNAs using deep sequencing data. Nucleic Acids Research, 42(D1), 68–73. https://doi.org/10.1093/nar/gkt1181
Laugesen, A., & Helin, K. (2014). Chromatin repressive complexes in stem cells, development, and cancer. Cell Stem Cell, 14(6), 735–751. https://doi.org/10.1016/j.stem.2014.05.006
Laugesen, A., Højfeldt, J. W., & Helin, K. (2019). Molecular mechanisms directing PRC2 recruitment and H3K27 methylation. Molecular Cell, 74(1), 8–18. https://doi.org/10.1016/j.molcel.2019.03.011.Molecular
Li, Y. (2021). Modern Epigenetics Methods in Biological Research Yuanyuan. Methods, 187, 104–113. https://doi.org/10.4049/jimmunol.18014
Lin, Z., Tang, X., Wan, J., Zhang, X., Liu, C., & Liu, T. (2021). Functions and mechanisms of circular RNAs in regulating stem cell differentiation. RNA Biology, 18(12), 2136–2149. https://doi.org/10.1080/15476286.2021.1913551
Liu, S., Zhou, J., Zhang, X., Liu, Y., Chen, J., Hu, B., Song, J., & Zhang, Y. (2016). Strategies to optimize adult stem cell therapy for tissue regeneration. International Journal of Molecular Sciences, 17(6), 1–16. https://doi.org/10.3390/ijms17060982
Loughran, S. J., Haas, S., Wilkinson, A. C., Klein, A. M., & Brand, M. (2020). Lineage commitment of hematopoietic stem cells and progenitors: insights from recent single cell and lineage tracing technologies. Experimental Hematology, 88, 1–6. https://doi.org/10.1016/j.exphem.2020.07.002
Ludikhuize, M. C., Meerlo, M., Gallego, M. P., Xanthakis, D., Burgaya Julià, M., Nguyen, N. T. B., Brombacher, E. C., Liv, N., Maurice, M. M., Paik, J. hye, Burgering, B. M. T., & Rodriguez Colman, M. J. (2020). Mitochondria Define Intestinal Stem Cell Differentiation Downstream of a FOXO/Notch Axis. Cell Metabolism, 32(5), 889-900.e7. https://doi.org/10.1016/j.cmet.2020.10.005
Maeng, Y. S., Kwon, J. Y., Kim, E. K., & Kwon, Y. G. (2015). Heterochromatin protein 1 alpha (HP1α: CBX5) is a key regulator in differentiation of endothelial progenitor cells to endothelial cells. Stem Cells, 33(5), 1512–1522. https://doi.org/10.1002/stem.1954
Mamidi, A., Prawiro, C., Seymour, P. A., de Lichtenberg, K. H., Jackson, A., Serup, P., & Semb, H. (2018). Mechanosignalling via integrins directs fate decisions of pancreatic progenitors. Nature, 564(7734), 114–118. https://doi.org/10.1038/s41586-018-0762-2
Mattout, A., Aaronson, Y., Sailaja, B. S., Raghu Ram, E. V., Harikumar, A., Mallm, J. P., Sim, K. H., Nissim-Rafinia, M., Supper, E., Singh, P. B., Sze, S. K., Gasser, S. M., Rippe, K., & Meshorer, E. (2015). Heterochromatin Protein 1β (HP1β) has distinct functions and distinct nuclear distribution in pluripotent versus differentiated cells. Genome Biology, 16(1), 7–9. https://doi.org/10.1186/s13059-015-0760-8
Mens, M. M. J., & Ghanbari, M. (2018). Cell Cycle Regulation of Stem Cells by MicroRNAs. Stem Cell Reviews and Reports, 14(3), 309–322. https://doi.org/10.1007/s12015-018-9808-y
Morgani, S., Nichols, J., & Hadjantonakis, A. K. (2017). The many faces of Pluripotency: In vitro adaptations of a continuum of in vivo states. BMC Developmental Biology, 17(1), 10–12. https://doi.org/10.1186/s12861-017-0150-4
Narayanan, K., Kumar, S., Padmanabhan, P., Gulyas, B., Wan, A., & Rajendran, V. (2018). Lineage-Specific Exosomes Could Override Extracellular Matrix Mediated Human Mesenchymal Stem Cell Differentiation. Biomate, 182, 312–322. https://doi.org/10.1016/j.biomaterials.2018.08.027.Lineage-Specific
Nichols, J., & Smith, A. (2009). Naive and Primed Pluripotent States. Cell Stem Cell, 4(6), 487–492. https://doi.org/10.1016/j.stem.2009.05.015
Novoseletskaya, E. S., Grigorieva, O. A., Efimenko, A. Y., & Kalinina, N. I. (2019). Extracellular Matrix in the Regulation of Stem Cell Differentiation. Biochemistry (Moscow), 84(3), 232–240. https://doi.org/10.1134/S0006297919030052
Nowell, C. S., & Radtke, F. (2017). Corneal epithelial stem cells and their niche at a glance. Journal of Cell Science, 130(6), 1021–1025. https://doi.org/10.1242/jcs.198119
O’Loghlen, A., Muñoz-Cabello, A. M., Gaspar-Maia, A., Wu, H. A., Banito, A., Kunowska, N., Racek, T., Pemberton, H. N., Beolchi, P., Lavial, F., Masui, O., Vermeulen, M., Carroll, T., Graumann, J., Heard, E., Dillon, N., Azuara, V., Snijders, A. P., Peters, G., Gil, J. (2012). MicroRNA regulation of Cbx7 mediates a switch of polycomb orthologs during ESC differentiation. Cell Stem Cell, 10(1), 33–46. https://doi.org/10.1016/j.stem.2011.12.004
Peng, H. & Abdel-Latif, A. (2019). Cellular Therapy for Ischemic Heart Disease: An Update (M. Z. Ratajczak, Ed.). Stem Cells, Advances in Experimental Medicine and Biology 1201., Springer Nature. https://doi.org/10.1007/978-3-030-31206-0_10
Prigione, A., & Adjaye, J. (2011). Modulation of mitochondrial biogenesis and bioenergetic metabolism upon in vitro and in vivo differentiation of human ES and iPS cells. International Journal of Developmental Biology, 54(11–12), 1729–1741. https://doi.org/10.1387/ijdb.103198ap
Rivera, L. B., & Bergers, G. (2014). Targeting vascular sprouts. Science, 344(6191), 1449–1450. https://doi.org/10.1126/science.1257071
Rocheteau, P., Gayraud-Morel, B., Siegl-Cachedenier, I., Blasco, M. A., & Tajbakhsh, S. (2012). A subpopulation of adult skeletal muscle stem cells retains all template DNA strands after cell division. Cell, 148(1–2), 112–125. https://doi.org/10.1016/j.cell.2011.11.049
Rodríguez-Colman, M. J., Schewe, M., Meerlo, M., Stigter, E., Gerrits, J., Pras-Raves, M., Sacchetti, A., Hornsveld, M., Oost, K. C., Snippert, H. J., Verhoeven-Duif, N., Fodde, R., & Burgering, B. M. T. (2017). Interplay between metabolic identities in the intestinal crypt supports stem cell function. Nature, 543(7645), 424–427. https://doi.org/10.1038/nature21673
Ryall, J. G., Cliff, T., Dalton, S., & Sartorelli, V. (2015). Metabolic Reprogramming of Stem Cell Epigenetics. Cell Stem Cell, 17(6), 651–662. https://doi.org/10.1016/j.stem.2015.11.012
Sancho, R., Cremona, C. A., & Behrens, A. (2015). Stem cell and progenitor fate in the mammalian intestine: Notch and lateral inhibition in homeostasis and disease. EMBO Reports, 16(5), 571–581. https://doi.org/10.15252/embr.201540188
Santanach, A., Blanco, E., Jiang, H., Molloy, K. R., Sansó, M., LaCava, J., Morey, L., & Di Croce, L. (2017). The Polycomb group protein CBX6 is an essential regulator of embryonic stem cell identity. Nature Communications, 8(1), 1–11. https://doi.org/10.1038/s41467-017-01464-w
Schofield R. (1978). The relationship between the spleen colony-forming cell and the haemopoietic stem cell. Blood cells, 4(1-2), 7–25.
Sellmer, A., Stangl, H., Beyer, M., Grünstein, E., Leonhardt, M., Pongratz, H., Eichhorn, E., Elz, S., Striegl, B., Jenei-Lanzl, Z., Dove, S., Straub, R. H., Krämer, O. H., & Mahboobi, S. (2018). Marbostat-100 Defines a New Class of Potent and Selective Antiinflammatory and Antirheumatic Histone Deacetylase 6 Inhibitors. Journal of Medicinal Chemistry, 61(8), 3454–3477. https://doi.org/10.1021/acs.jmedchem.7b01593
Seo, B. J., Yoon, S. H., & Do, J. T. (2018). Mitochondrial dynamics in stem cells and differentiation. International Journal of Molecular Sciences, 19(12). https://doi.org/10.3390/ijms19123893
Shahbazian, M. D., & Grunstein, M. (2007). Functions of site-specific histone acetylation and deacetylation. Annual Review of Biochemistry, 76, 75–100. https://doi.org/10.1146/annurev.biochem.76.052705.162114
Shapiro, I. M., Landis, W. J., & Risbud, M. V. (2015). Matrix vesicles: Are they anchored exosomes? Bone, 79, 29–36. https://doi.org/10.1016/j.bone.2015.05.013
Spit, M., Koo, B. K., & Maurice, M. M. (2018). Tales from the crypt: Intestinal niche signals in tissue renewal, plasticity and cancer. Open Biology, 8(9). https://doi.org/10.1098/rsob.180120
Sugawara, K., Tsuruta, D., Ishii, M., Jones, J. C. R., & Kobayashi, H. (2008). Laminin-332 and -511 in skin. Experimental Dermatology, 17(6), 473–480. https://doi.org/10.1111/j.1600-0625.2008.00721.x
Swift, J., Ivanovska, I. L., Buxboim, A., & Harada, T. (2013). Nuclear Lamin-A Scales with Tissue Stiffness and Enhances Matrix-Directed Differentiation. Science, 341(6149), 1240104. https://doi.org/10.1126/science.1240104.Nuclear
Tatavosian, R., Kent, S., Brown, K., Yao, T., Duc, H. N., Huynh, T. N., Zhen, C. Y., Ma, B., Wang, H., & Ren, X. (2019). Nuclear condensates of the Polycomb protein chromobox 2 (CBX2) assemble through phase separation. Journal of Biological Chemistry, 294(5), 1451–1463. https://doi.org/10.1074/jbc.RA118.006620
Thomas, D., O’Brien, T., & Pandit, A. (2018). Toward Customized Extracellular Niche Engineering: Progress in Cell-Entrapment Technologies. Advanced Materials, 30(1), 1–20. https://doi.org/10.1002/adma.201703948
Tyurin-Kuzmin, P. A., Molchanov, A. Y., Chechekhin, V. I., Ivanova, A. M., & Kulebyakin, K. Y. (2020). Metabolic Regulation of Mammalian Stem Cell Differentiation. Biochemistry (Moscow), 85(3), 264–278. https://doi.org/10.1134/S0006297920030025
Urbán, N., & Cheung, T. H. (2021). Stem cell quiescence: the challenging path to activation. Development (Cambridge, England), 148(3), dev165084. https://doi.org/10.1242/dev.165084
van Wijnen, A. J., Bagheri, L., Badreldin, A. A., Larson, A. N., Dudakovic, A., Thaler, R., Paradise, C. R., & Wu, Z. (2021). Biological functions of chromobox (CBX) proteins in stem cell self-renewal, lineage-commitment, cancer and development. Bone, 143, 115659. https://doi.org/10.1016/j.bone.2020.115659
Varum, S., Rodrigues, A. S., Moura, M. B., Momcilovic, O., Easley IV, C. A., Ramalho-Santos, J., van Houten, B., & Schatten, G. (2011). Energy metabolism in human pluripotent stem cells and their differentiated counterparts. PLoS ONE, 6(6). https://doi.org/10.1371/journal.pone.0020914
Wang, Y., & Blelloch, R. (2011). Cell Cycle Regulation by microRNAs in Stem Cells. Cell Cycle, 53, 459–472. https://doi.org/10.1007/978-3-642-19065-0
Wu, J. Y., Wu, S. N., Zhang, L. P., Zhao, X. S., Li, Y., Yang, Q. Y., Yuan, R. Y., Liu, J. L., Mao, H. J., & Zhu, N. W. (2022). Stem Cell-Derived Exosomes: A New Method for Reversing Skin Aging. Tissue Engineering and Regenerative Medicine, 19(5), 961–968. https://doi.org/10.1007/s13770-022-00461-5
Yao, Y., Chen, R., Wang, G., Zhang, Y., & Liu, F. (2019). Exosomes derived from mesenchymal stem cells reverse EMT via TGF-β1/Smad pathway and promote repair of damaged endometrium. Stem Cell Research and Therapy, 10(1), 1–17. https://doi.org/10.1186/s13287-019-1332-8
Yi, S., Ding, F., Gong, L., & Gu, X. (2017). Extracellular Matrix Scaffolds for Tissue Engineering and Regenerative Medicine. Current Stem Cell Research & Therapy, 12(3), 233–246. https://doi.org/10.2174/1574888x11666160905092513
Zakrzewski, W., Dobrzyński, M., Szymonowicz, M., & Rybak, Z. (2019). Fuel Cells: Past, Present and Future. Stem Cell Research and Therapy, 128(5), 329–332. https://doi.org/10.1541/ieejfms.128.329
Zhang, X., Zhang, S., & Wang, T. (2022). How the mechanical microenvironment of stem cell growth affects their differentiation: a review. Stem Cell Research and Therapy, 13(1), 1–17. https://doi.org/10.1186/s13287-022-03070-0
Zheng, L., Roeder, R. G., & Luo, Y. (2003). S Phase Activation of the Histone H2B Promoter by OCA-S, a Coactivator Complex that Contains GAPDH as a Key Component an S phase-specific cofactor(s) or modification of the general transcription machinery in conferring S phase-specific H2B transcription. . Cell, 114, 255–266.
Visual Sources
Cover Image: "Mesenchymal stem cell". (n.d., 2023). [Illustration]. DVCStem. https://www.dvcstem.com/post/stem-cell-differentiation
Figure 1: Potential fates of a stem cell. (n.d.). [Illustration]. Cusabio. https://www.cusabio.com/cytokines/Cell-Differentiation.html
Figure 2: Oct4/Sox2/Nanog form a core transcriptional network regulating the stem cell machinery. Bosnali, M., Münst, B., Thier, M., & Edenhofer, F. (2009). [Illustration]. Deciphering the stem cell machinery as a basis for understanding the molecular mechanism underlying reprogramming. Cellular and Molecular Life Sciences, 66(21), 3403–3420. https://doi.org/10.1007/s00018-009-0095-2
Figure 3: The PcG system dynamically regulates developmental factors during cell differentiation. Roure, V., & Bantignies, F. (2009). [Illustration]. Polycomb group-mediated gene silencing mechanisms: Stability versus flexibility. Epigenomics, 1(2), 301–318. https://doi.org/10.2217/EPI.09.28
Figure 4: Summary of Cbx7s mechanism in embryonic stem cells. (OLoghlen & Gil, 2011). [Illustration]. Atlas Genet Cytogenet Oncol Haematol. https://atlasgeneticsoncology.org/gene/43845/cbx7-%28chromobox-homolog-7%29
Figure 5: How oxygen levels influence ESCs and iPSCs. Nit, K., Tyszka-Czochara, M., & Bobis-Wozowicz, S. (2021). [Illustration]. Oxygen as a Master Regulator of Human Pluripotent Stem Cell Function and Metabolism. Journal of Personalized Medicine, 11(9), 905. https://doi.org/10.3390/jpm11090905
Figure 6: Impact of oxygen on the differentiation of hPSCs. Nit, K., Tyszka-Czochara, M., & Bobis-Wozowicz, S. (2021). [Illustration]. Oxygen as a Master Regulator of Human Pluripotent Stem Cell Function and Metabolism. Journal of Personalized Medicine, 11(9), 905. https://doi.org/10.3390/jpm11090905
Figure 7: Stem cell metabolism is dynamically modulated to control stemness, proliferation, and cell commitment. Rigaud, V. O. C., Hoy, R., Mohsin, S., & Khan, M. (2020). [Illustration]. Stem Cell Metabolism: Powering Cell-Based Therapeutics. Cells, 9(11). https://doi.org/10.3390/cells9112490
Figure 8: Stem cell metabolism during lineage commitment and reprogramming. Ryall, J. G., Cliff, T., Dalton, S., & Sartorelli, V. (2015). [Illustration]. Metabolic Reprogramming of Stem Cell Epigenetics. Cell Stem Cell, 17(6), 651–662. https://doi.org/10.1016/j.stem.2015.11.012
Figure 9: The features and molecular regulation of adult stem cell quiescence and activation. Urbán, N., & Cheung, T. H. (2021). [Illustration]. Stem cell quiescence: the challenging path to activation. Development (Cambridge, England), 148(3), dev165084. https://doi.org/10.1242/dev.165084
Figure 10: The perivascular stem cell niche and its influence. Perottoni, S., Neto, N. G. B., Di Nitto, C., Dmitriev, R. I., Raimondi, M. T., & Monaghan, M. G. (2021). [Illustration]. Intracellular label-free detection of mesenchymal stem cell metabolism within a perivascular niche-on-a-chip. Lab on a Chip, 21(7), 1395–1408. https://doi.org/10.1039/d0lc01034k
Figure 11: Cell interactions in a biological system. Thomas, D., O’Brien, T., & Pandit, A. (2018). [Illustration]. Toward Customized Extracellular Niche Engineering: Progress in Cell-Entrapment Technologies. Advanced Materials, 30(1), 1–20. https://doi.org/10.1002/adma.201703948
Figure 12: The schematic diagram of extracellular matrix scaffolds. Yi, S., Ding, F., Gong, L., & Gu, X. (2017). [Illustration]. Extracellular Matrix Scaffolds for Tissue Engineering and Regenerative Medicine. Current Stem Cell Research & Therapy, 12(3), 233–246. https://doi.org/10.2174/1574888x11666160905092513
Figure 13: Differentiation of mouse pancreatic progenitors into either endocrine or duct cells depends on the ECM composition and mechanical properties. Novoseletskaya, E. S., Grigorieva, O. A., Efimenko, A. Y., & Kalinina, N. I. (2019). [Illustration]. Extracellular Matrix in the Regulation of Stem Cell Differentiation. Biochemistry (Moscow), 84(3), 232–240. https://doi.org/10.1134/S0006297919030052
Figure 14: Role of ECM components and structure in the regulation of proliferation and differentiation of human limbal stem cells. Novoseletskaya, E. S., Grigorieva, O. A., Efimenko, A. Y., & Kalinina, N. I. (2019). [Illustration]. Extracellular Matrix in the Regulation of Stem Cell Differentiation. Biochemistry (Moscow), 84(3), 232–240. https://doi.org/10.1134/S0006297919030052
Comments