Stem Cell Biotechnology Series: Induced Pluripotent Stem Cells (iPSCs) and Genetic Engineering
- Victor Cornily
- Sep 24, 2023
- 26 min read
Updated: Sep 11, 2024
Foreword
The field of stem cells is brimming with promise and intrigue. As the understanding of these remarkable foundational components of life continues to evolve, so does the capacity to harness their extraordinary potential. Stem cells have enchanted the minds of scientists, medical professionals, and curious individuals alike, presenting unparalleled opportunities to revolutionise healthcare and regenerative medicine. This series of articles aims to unravel the mysteries of stem cell differentiation, explore the mechanisms governing their self-renewal, and examine the factors that shape their destiny. Within these articles, readers may discover the immense potential of induced pluripotent stem cells (iPSCs) in reshaping personalised medicine while uncovering their awe-inspiring regenerative capabilities. Moreover, this series sheds light on the critical challenges and limitations inherently intertwined with this field, from ensuring the safety and efficacy of stem cell therapies through navigating the complex terrain of regulatory frameworks and public perception to debating ethical uncertainties.
This series is divided into eight articles:
5. Stem Cell Biotechnology Series: Induced Pluripotent Stem Cells (iPSCs) and Genetic Engineering
Stem Cell Biotechnology Series: Induced Pluripotent Stem Cells (iPSCs) and Genetic Engineering
In contemporary biotechnology, induced pluripotent stem cells (iPSCs) and genetic engineering have emerged as main forces, reshaping the landscape of stem cell research. Genetic engineering, a cornerstone of modern biotechnology, involves deliberately manipulating an organism's genetic material to achieve specific outcomes. Within the context of iPSC research, genetic engineering plays a crucial role in reprogramming somatic cells into pluripotent stem cells. The convergence of iPSCs with cellular reprogramming and precise genomic manipulation holds extraordinary promise for regenerative medicine, disease modelling, and therapeutic advancements. This article delves into the sophisticated relationship between iPSCs and genetic engineering, dissecting the tools, techniques, and transformative potential of their conjunction.
IPSCs: Historic Summary
Induced pluripotent stem cells symbolise an influential breakthrough in stem cell biology, offering a versatile and ethically sound alternative to traditional embryonic stem cells. The advent of iPSCs can be traced back to pioneering work conducted by Gurdon et al. in 1958, which laid the foundation for cellular reprogramming through somatic cell nuclear transfer. Gurdon's experiments involved transplanting the nuclei of mature intestinal epithelial cells into enucleated frog eggs, resulting in the development of healthy tadpoles. This work laid the groundwork for understanding pluripotency-inducing factors within embryonic cells, setting the stage for ensuing developments.
In 2006, Yamanaka and Takahashi ushered in a new era with their groundbreaking discovery of iPSCs through retroviral-mediated expression of four key genes, Oct4, Sox2, Klf4, and c-Myc (collectively known as the "Yamanaka Factors" or OSKM factors). This climactic discovery marked the inception of iPSCs, a distinct avenue to pluripotency. Building on the lessons from Gurdon's experiments, Yamanaka and Takahashi's work showcased the potential to directly reprogram somatic cells into a pluripotent state, for which they received the Nobel prize (The Nobel Prize, 2012). These historical events represent a critical juncture in scientific progress, with far-reaching implications for the future of healthcare and scientific inquiry. With the arrival of iPSCs, an inevitable revolution began - regenerative medicine and cellular research gained a rich source of pluripotent cells for many applications. Figure 1 illustrates the most relevant historical advances in this field.
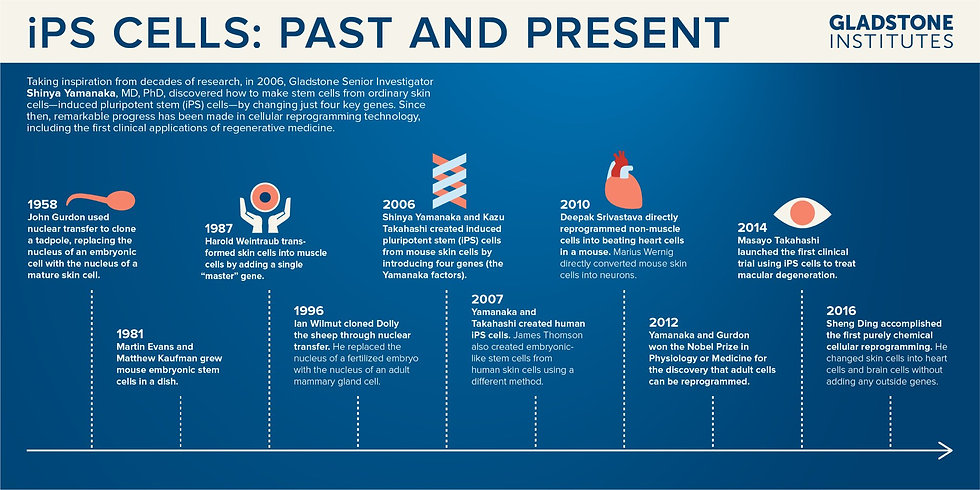
What Are Induced Pluripotent Stem Cells?
To reiterate knowledge from previous articles in this series, iPSCs are a type of pluripotent stem cells, meaning they possess the remarkable capacity to differentiate into virtually any cell type found in the human body, including neurons, cardiomyocytes, hepatocytes, and more. This inherent versatility positions iPSCs as an adaptable and ethically sound alternative to embryonic stem cells. The foundation of iPSC technology is the process of cellular reprogramming. It involves taking somatic cells, such as skin fibroblasts or blood cells, and rewinding their developmental clock, transforming them into a state resembling embryonic stem cells. The potential applications of iPSCs are vast and multifaceted. They hold immense promise in regenerative medicine, where they can be used to generate patient-specific tissues and organs for transplantation, potentially mitigating the shortage of donor organs and reducing the risk of transplant rejection.
Additionally, iPSCs provide a powerful platform for disease modelling. By reprogramming cells from patients with genetic disorders or complex diseases, researchers can create in vitro models that accurately mimic the disease's progression, enabling the development of targeted therapies and personalised medicine approaches. iPSCs have also opened new frontiers in drug discovery and toxicity testing. Pharmaceutical companies are increasingly using iPSC-derived cells to screen potential drug candidates and assess their safety profiles, reducing the need for animal testing and accelerating the drug development process. iPSCs also offer insights into developmental biology, as they enable the study of human embryonic development and differentiation in previously inaccessible ways. One of the significant advantages of iPSCs is their ethical acceptability, which will be discussed in more depth later in the series.
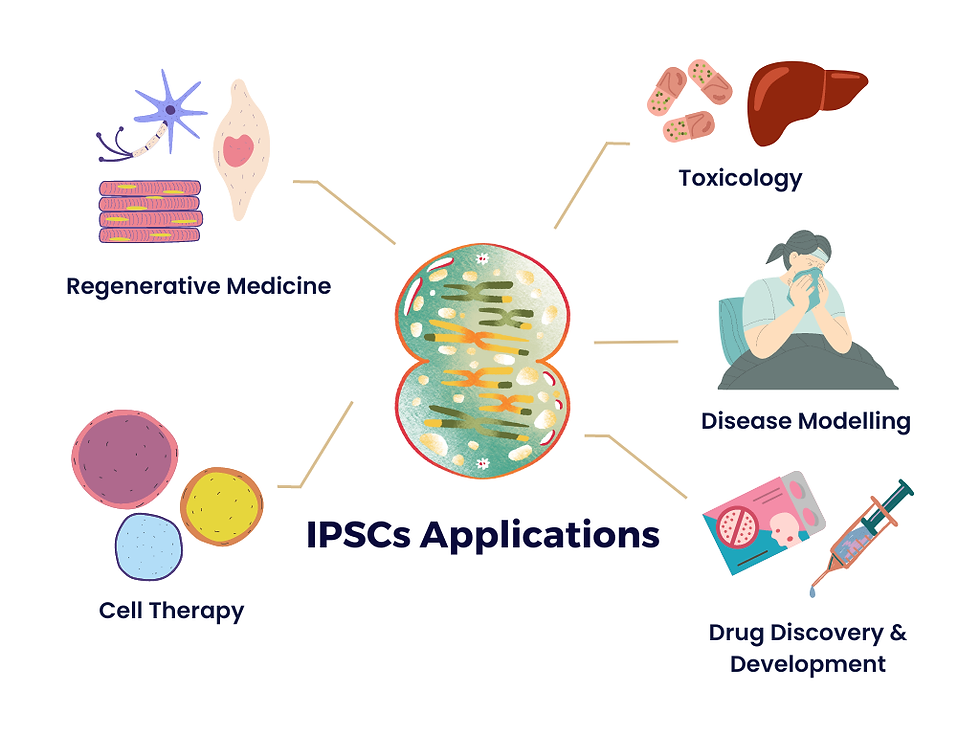
Reprogramming The Cell
A diverse array of somatic cell types, ranging from fibroblasts and blood cells to keratinocytes, liver, gastrointestinal cells, and even cancer cells, has been harnessed as sources for deriving iPSCs. The initial proof-of-concept studies that aimed to create iPSCs resembling mouse embryonic stem cells were primarily centred on the retroviral transduction of mouse fibroblasts using four specific transcription factors. To date, diverse methodologies have emerged, each finely tuned to meet specific requirements. Remarkably, the most valuable techniques for investigating human diseases and advancing therapeutic applications strike a delicate balance between efficiency and adaptability. They excel in generating iPSCs from sources that may have limited availability, possess the versatility to reprogram different types of somatic cells and operate without leaving a genetic footprint (Menon et al., 2016). These rigorous criteria have spurred the development and widespread adoption of multiple reprogramming techniques [Figure 3].
Somatic cells - specialised cells with a specific function - can be reprogrammed to regain pluripotency, a state similar to embryonic stem cells. This reprogramming is typically achieved by introducing a combination of transcription factors, such as OSKM, into the somatic cells. These factors act as molecular switches, turning off the somatic cell's specific gene expression patterns and reactivating genes associated with pluripotency. As a result, the somatic cells undergo a profound transformation, resetting their identity to become iPSCs capable of self-renewal and differentiation into a wide range of cell types (Liu et al., 2013).

Although its presence is not a strict necessity, c-Myc, previously deemed indispensable, has been found to enhance reprogramming efficiency. (Wernig et al., 2008). A comprehensive study conducted in 2009 shed light on the nuanced roles of these four reprogramming factors. The group observed that ectopic (out-of-place) expression of c-Myc, when expressed individually in fibroblasts, primarily promotes an ESC-like gene expression pattern, mainly acting in the initial phases of reprogramming. In contrast, Oct4, Sox2, and Klf4 come into play later, re-establishing an ESC-like gene expression profile and activating other pluripotency genes as reprogramming progresses. Incompletely reprogrammed cells, lacking these coordinated activities, may signify incomplete epigenetic transformations or the necessity for additional factors to cooperatively bind target genes with Oct4, Sox2, and Klf4 (Sridharan et al., 2009).
While the reprogramming of iPSCs was initially orchestrated using a quartet of transcription factors, namely Oct4, Sox2, Klf4, and c-Myc, the following research has elucidated their interchangeability with other factors. A research group demonstrated successful human fibroblast reprogramming using Oct4, Sox2, Nanog and Lin28, revealing that Klf4 and c-Myc can be substituted (Yu & Thomson, 2008). Some later breakthroughs have shown that alternative factors can replace Klf4 in mouse iPSC reprogramming. For instance, the orphan nuclear receptor Esrrb was found to effectively replace Klf4 when co-transduced with either OSM or OS factors (Feng et al., 2009). Intriguingly, three of the reprogramming factors, namely Sox2, Nanog, and Esrrb, are physically associated with Oct4, suggesting that the activation of pluripotency-associated transcriptional pathways may necessitate the concurrent presence of Oct4 and its linked factors for precise interactions at shared targets (Liang et al., 2008).
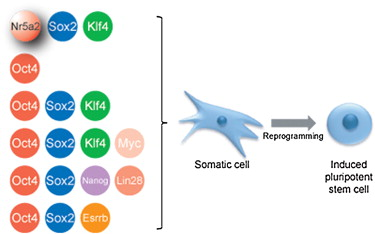
Early iPSC Generation: Viral Transduction Methods
As mentioned, the initial generation of iPSCs marked a significant milestone in regenerative medicine. This achievement was accomplished through a technique known as retroviral transduction, where the OSKM factors (Oct4, Sox2, Klf4, and c-Myc) were introduced into mouse fibroblasts (Takahashi & Yamanaka, 2006). Retroviral transduction has proven successful in reprogramming various cell types, including mouse and human fibroblasts, neural stem cells, keratinocytes, adipose cells, liver cells, and blood cells. However, it is essential to note that the reprogramming efficiency with human cells using this method ranges between 0.01% to 0.02% (Takahashi et al., 2007). An alternative approach to transducing OSKM factors for iPSC derivation involves using a lentiviral system, which boasts a higher efficiency rate ranging from 0.1% to 2% compared to retroviral transduction (González et al., 2011).
While the discovery of the Yamanaka factors and their application through retroviral and lentiviral systems provided promising means to obtain embryonic-like stem cells in abundant quantities, it came with consequential drawbacks. These disadvantages include the integration of viral DNA into the host genome and concerns about the potential pro-cancerous role of c-Myc in malignant transformation, which limits the translational applications of iPSC lines derived using these methods (Lee et al., 2023). Consequently, from a clinical standpoint, direct reprogramming through retroviral and lentiviral transduction of OSKM factors is not considered a fully translational approach, and alternative reprogramming methods have earned popularity (Menon et al., 2016).
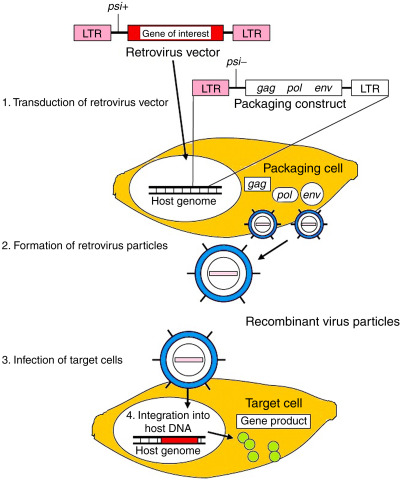
Non-Integrating DNA-Based Methods
Utilising retroviral transduction as an early method for reprogramming somatic cells into iPSCs posed a substantial challenge due to the integration of viral vectors into the host genome. This integration raised concerns about the potential disruption of endogenous genes and the stability of resulting iPSCs, limiting their suitability for clinical applications. (Nakagawa et al., 2008). The integration of viral vectors into the genome can result in insertional mutagenesis, interference with gene transcription, genome instability, and even the induction of malignant transformation. For instance, studies in mice showed that iPSC-derived chimaeras often developed tumours due to the reactivation of oncogenes like c-Myc (Markoulaki et al., 2009). Although there have been efforts to generate iPSCs without c-Myc, the integrated reprogramming factors themselves could also pose risks (Wernig et al., 2008).
Recognising the need for safer reprogramming methods, researchers have developed non-integrating strategies. The fundamental principle of these methods is to avoid the risks associated with viral integration (Wu & Izpisua Belmonte, 2015). One approach involves doxycycline (DOX)-inducible lentiviral vectors with LoxP sites, allowing for the excision of transgenes using Cre-recombinase (Chang et al., 2009). Another technique utilises piggyback transposons, mobile genetic elements that can efficiently integrate into TTAA sequence chromosomal sites without full integration (Woodard & Wilson, 2015). Replication-defective adenoviral vectors are another non-integrating option. However, optimising their efficiency in reprogramming is necessary for widespread use in translational medicine (Tasca et al., 2020). Non-viral minicircle DNA vectors have been explored, but further validation is required for translational studies (W. Zhou & Freed, 2009). Episomal plasmids provide another avenue for non-integrating reprogramming, offering potential for future applications despite low efficiency (Hu et al., 2016). Although these methods have shown promise, ensuring the complete removal of residual vector sequences before clinical applications is essential.

Non-Integrating Non-DNA-Based Methods
Subsequent advancements have been achieved in the pursuit of methodologies devoid of DNA involvement. One of these promising alternatives is the use of Sendai virus (SV), a single-stranded RNA virus. Unlike retroviruses and lentiviruses, SV does not introduce genomic material into the host cell nucleus. This feature, coupled with the ability to remove SV using antibody-mediated negative selection easily, makes it an attractive choice for generating iPSCs. Research conducted by Fusaki et al. demonstrated the successful induction of human iPSCs from fibroblasts using SV, offering a safe alternative to integrating techniques. Notably, the efficiency of iPSC derivation with SV is approximately 0.1%, which rivals lentiviral methods while maintaining a non-integrating characteristic. Although working with SV can be more challenging compared to lentiviruses, the availability of commercially prepared Sendai viral extracts has made this approach increasingly promising for translational research (Fusaki et al., 2009).
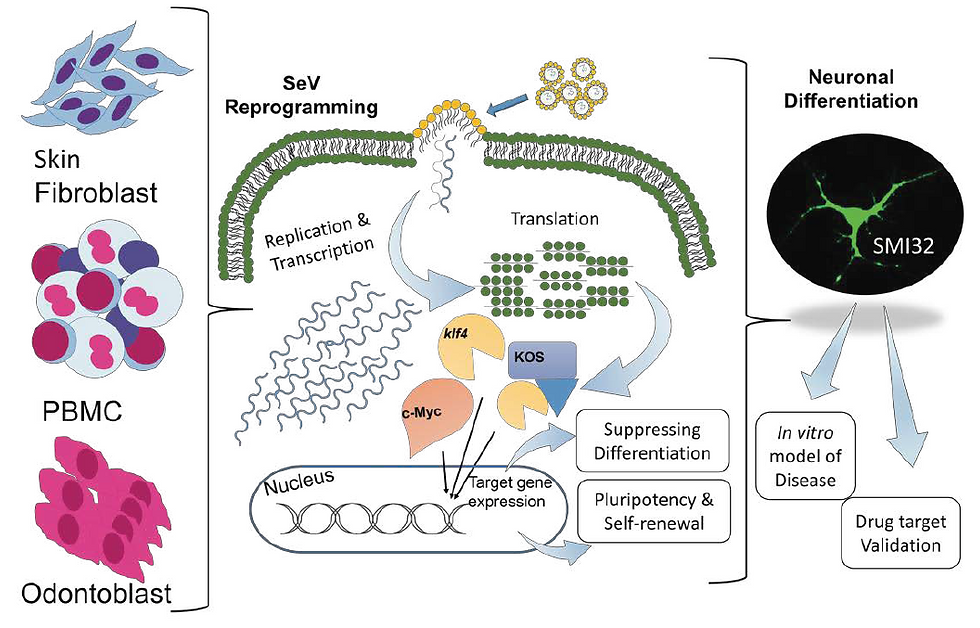
Another advanced method for converting somatic cells into iPSCs without introducing genetic material is through mRNA transfection, known as RNA-induced pluripotent stem cells (RiPSCs). This safe and effective approach creates iPSCs with minimal genetic changes, often called a "null footprint." RiPSCs also trigger a weaker immune response, which is beneficial for potential medical applications (Liu & Verma, 2015). It has been shown that by repeatedly introducing synthetic modified messenger RNAs carrying the OSKM factors, designed to bypass natural antiviral responses, they can efficiently reprogram various human cells into pluripotent ones. This includes cells from human keratinocytes, BJ human neonatal fibroblasts, MRC-5 human fetal lung fibroblasts, and fibroblasts from cystic fibrosis patients. However, it is important to note that this method can be time-consuming and labour-intensive (Warren et al., 2010).
Researchers have harnessed the potential of purified OSKM proteins for reprogramming by exploring yet another innovative approach. Kim and colleagues (2009) demonstrated the generation of stable iPSCs from human fibroblasts by directly delivering four reprogramming protein factors, achieving an efficiency of 0.001%. These iPSC lines, called p-iPSCs, were successfully maintained for over 35 passages and could be differentiated into various cell types, reflecting their pluripotency. However, a significant challenge of this procedure lies in delivering OSKM proteins into cells efficiently, as they have limited ability to cross cellular membranes. This hurdle has been addressed by fusing the proteins with short basic segments, such as cell-penetrating peptides (CPPs), containing a high proportion of basic amino acids like arginine and lysine. Several studies have reported successful reprogramming using CPP-anchored reprogramming OSKM proteins, eliminating the need for genetic manipulation or chemical treatments (Frøslev et al., 2022). Nonetheless, it is critical to note that despite the induction of pluripotency, bioactive reprogramming proteins are challenging to produce in large quantities, and their efficiency typically ranges from 0.001% to 4%.
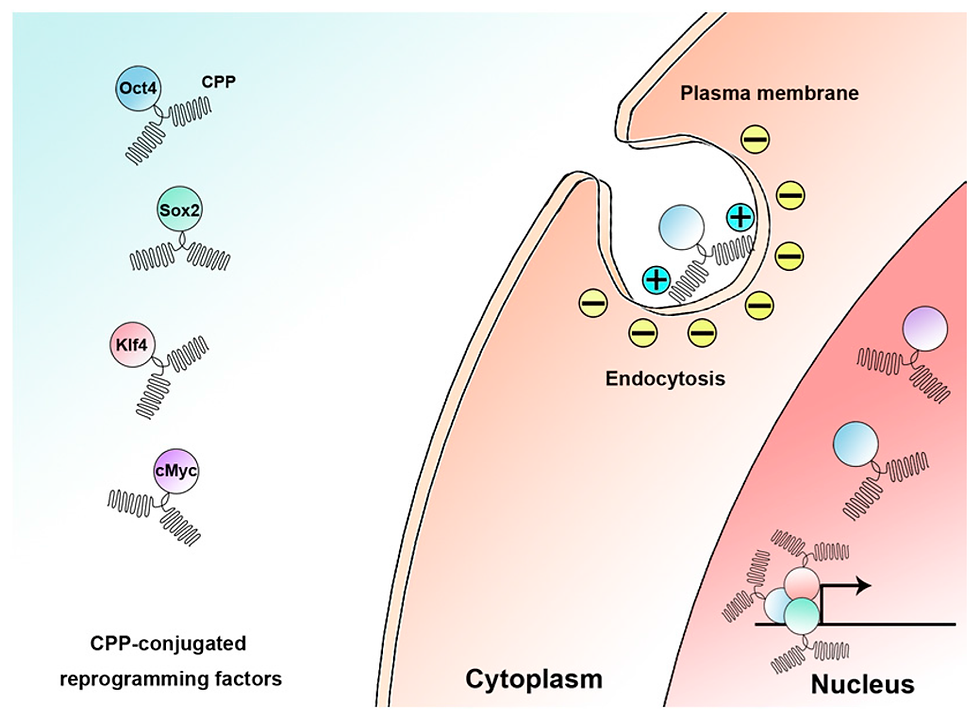
Additional Factors Influencing Reprogramming Efficiency
The slow and inefficient nature of the reprogramming process presents significant limitations for scientific investigations and potential clinical applications. Researchers have explored various strategies to boost reprogramming efficiency to overcome these challenges. One approach involves introducing additional factors, like SV40 large T antigen and human telomerase, which have been shown to enhance the reprogramming of human fibroblasts when combined with specific factors (Mali et al., 2008). The advantage of these enhancements is that they do not integrate foreign genetic material into the iPSCs (Park et al., 2008). Manipulating DNA methyltransferase levels by inhibiting or knocking down the enzyme has been shown to enhance reprogramming (Mikkelsen et al., 2008). Furthermore, a combination of interventions, including p53 knockdown and the introduction of UTF1, has led to a remarkable 100-fold increase in reprogramming efficiency in human fibroblasts. UTF1, regulated by Oct4-Sox2, activates genes crucial for reprogramming, while p53 inhibition enhances cell survival but raises concerns about potential genomic abnormalities in the resulting iPSCs (Sarig et al., 2010).
Although transcription factors and epigenetic modifiers have garnered significant attention for their roles in reprogramming, research has highlighted the importance of additional key players (Ivey et al., 2008). A class of small non-coding RNAs, miRNAs, appear to be crucial regulators of reprogramming. These molecules, known for their roles in maintaining the pluripotent state of embryonic stem cells, are now recognised for their potential impact on reprogramming efficiency. One notable miRNA is mir-302, abundantly expressed in human ESCs. This miRNA can remarkably convert human cancer cells into cells that closely resemble ESCs (Lin et al., 2020). This discovery stresses the importance of miRNAs in mediating the transition from a somatic cell state to a pluripotent state. However, the precise mechanisms and full extent of miRNA involvement in reprogramming are subjects of ongoing research.
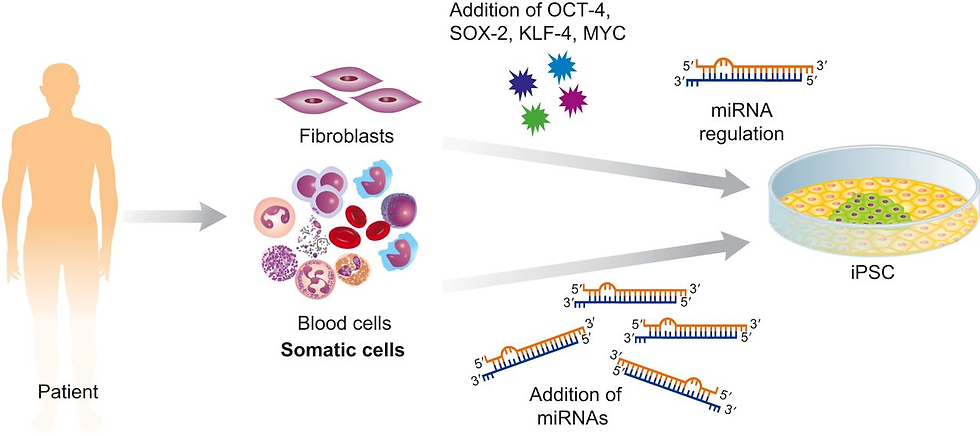
Small molecules represent another facet of reprogramming strategies. They have attracted attention for their ability to enhance reprogramming efficiency when combined with conventional reprogramming factors. Notable examples of such molecules include 5-aza-cytidine (AZA), valproic acid (VPA), and BIX-01294 (BIX) (Feng et al., 2009).
AZA operates as a DNA methyltransferase inhibitor, pivotal in DNA demethylation. DNA methylation means adding methyl groups to cytosine bases within CpG dinucleotides, regulating gene expression. AZA functions by mimicking the structure of DNA building blocks and becoming incorporated into the DNA during replication. Once integrated, AZA forms irreversible covalent bonds with DNA methyltransferases, the enzymes responsible for methyl group addition. These bonds create trapped complexes, rendering the methyltransferases inactive and preventing further methylation during subsequent DNA replication and repair cycles. Consequently, DNA methylation levels decrease at specific genomic regions, including gene promoters. This demethylation process can reactivate genes that were previously silenced. In the context of reprogramming partially reprogrammed cells into iPSCs, AZA's demethylating action is important for reactivating pluripotency-related genes like Oct4, facilitating the transition to a fully pluripotent state and the successful generation of iPSCs (Sellers et al., 2018).
VPA boosts reprogramming efficiency by altering histone acetylation and overall gene expression patterns. Histone acetylation, an essential epigenetic modification, influences chromatin structure by relaxing it, thus making the underlying DNA more accessible to transcription factors and the transcriptional machinery. As a histone deacetylase inhibitor, VPA intervenes in this process, preventing the removal of acetyl groups from histone proteins. Consequently, VPA induces a state of histone hyperacetylation within the cell, fostering a chromatin structure that is more permissive to gene activation. This alteration in chromatin structure has a profound impact on the reprogramming process (Leo et al., 2023).
Pluripotency genes are activated more rapidly when VPA is introduced. The expedited activation of these markers accelerates the transition of somatic cells to a pluripotent state. Moreover, VPA's influence on gene expression can reduce the reliance on some of the reprogramming factors traditionally used in the process. As aforementioned, standard reprogramming protocols introduce a set of transcription factors, such as OSKM, to induce pluripotency. However, VPA's epigenetic modifications and the resulting changes in gene expression may alleviate the need for all of these factors. This streamlined approach not only simplifies the reprogramming process but also minimises the genetic manipulations required (Zeng et al., 2020).
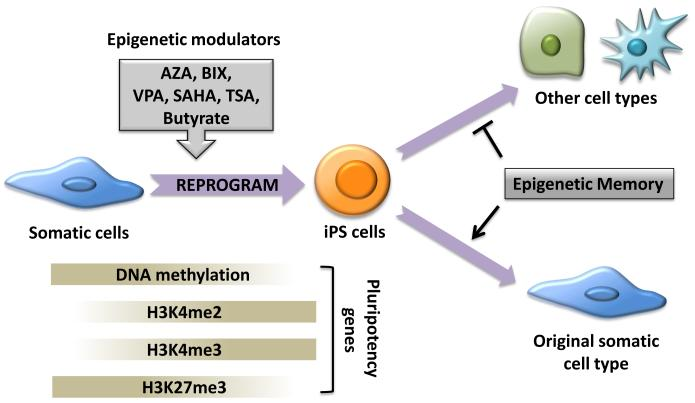
BIX acts as an inhibitor of histone lysine methyltransferases, with a notable focus on G9a histone methyltransferase. Histone methyltransferases are critical epigenetic regulators responsible for the addition of methyl groups to specific lysine residues on histone proteins, a process that profoundly impacts gene expression. BIX enhances the expression of pluripotency markers such as Oct4, Sox2, and Nanog. Secondly, BIX facilitates the erasure of epigenetic marks associated with the cell's somatic identity by inhibiting G9a-mediated histone methylation, a modification often correlated with gene silencing. This inhibition promotes the opening of chromatin structure, rendering DNA more accessible to transcription factors responsible for the activation of pluripotency-associated genes (Epsztejn-Litman et al., 2008). In addition, BIX significantly augments the overall efficiency of cellular reprogramming. Somatic cells subjected to BIX treatment demonstrate an increased likelihood of successfully transitioning to a pluripotent state and becoming iPSCs. However, it is noteworthy to underscore that BIX is typically employed in conjunction with other factors and reprogramming protocols to achieve optimal outcomes (Shi et al., 2008). The precise acting mechanisms of these small molecules are still under study, but their significance in reshaping the epigenetic landscape during reprogramming is evident.
Manipulating signalling pathways, including Wnt, MEK/ERK, GSK3, and LIF, also partakes in regulating reprogramming (Silva & Smith, 2008). The combination of MEK and GSK3 inhibition, known as "2i," promotes an ESC-like ground state and the transition from pre-iPSCs to fully competent iPSCs. This inhibition prevents lineage commitment induced by the ERK signalling pathway, ensuring the maintenance of self-renewal. The canonical Wnt signalling pathway, activated by a Wnt3a-conditioned medium, enhances reprogramming efficiency, potentially through factors like c-Myc and Tcf3 (Cole et al., 2008).
The ability of transcription factors alone to induce pluripotency was a remarkable discovery and underscored the dominant role of core reprogramming factors in reprogramming, potentially even overshadowing epigenetics’ influence (Niwa, 2007). It is worth mentioning that pluripotent stem cells have unique epigenetic features. They have many marks on their chromatin that signal active genes and very few that silence genes. When these stem cells start to change into specialised cells during differentiation, there are alterations in how genes are marked on the chromatin. Therefore, it makes it harder for genes to be turned on, leading to a less ‘transcriptionally permissive’ state, which means that genes are not as actively read and used (Meshorer & Misteli, 2006).
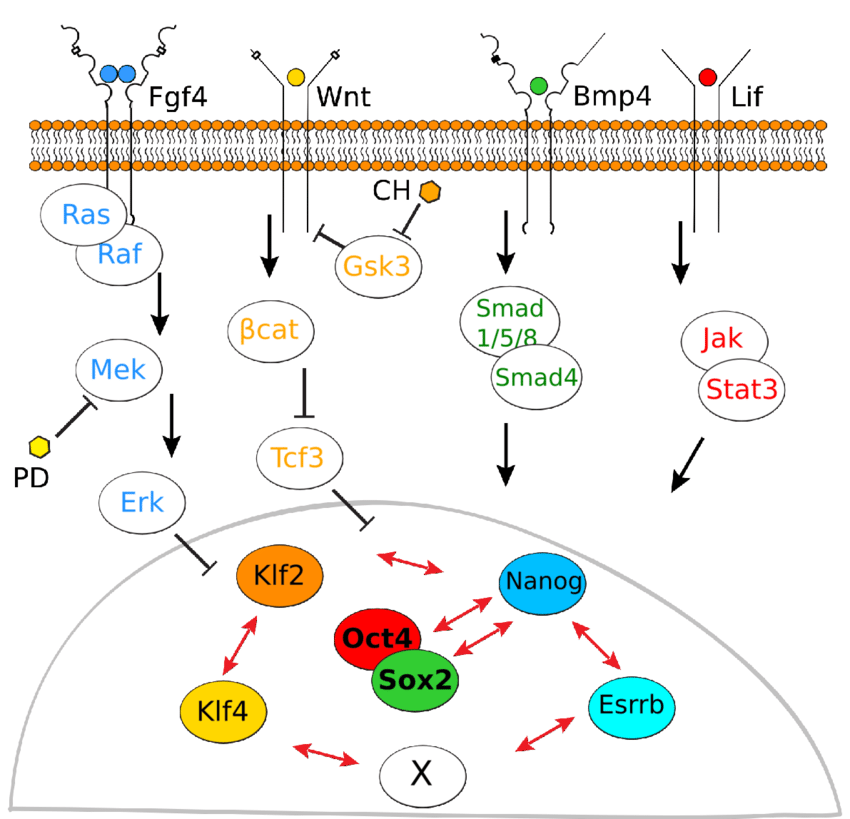
CRISPR/Cas Meets iPSCs
The fusion of genome editing techniques with the remarkable potential of stem cells represents a climacteric convergence in scientific exploration and therapeutic innovation, with iPSCs at the forefront of this journey. Over the last decade, the field of genome editing has burgeoned, propelled by the discovery of versatile and user-friendly tools, with the CRISPR/Cas system leading the charge. Concurrently, advances in in vitro culture systems, particularly the development of human stem cell-derived organoids, have provided experimental models that increasingly mirror the complexity of human organs (Liu et al., 2018). As these two transformative technologies intersect, they promise to unlock profound insights into fundamental biological processes unique to iPSCs, such as pluripotency and differentiation. Moreover, CRISPR-based therapeutic strategies have emerged within the context of iPSCs, encompassing both the transplantation of ex vivo genetically modified stem cells and direct in vivo genome targeting for regenerative medicine and disease modelling. This collision of cutting-edge stem cell cultures and CRISPR-based genome editing heralds a new era in science and medicine (Benabdellah et al., 2020).
Different CRISPR/Cas system types have been discovered, but none has made as significant an impact in human cells as CRISPR/Cas9 (Hendriks et al., 2020). In iPSCs, CRISPR/Cas9 operates through a simple yet powerful two-component system comprising a guide RNA (gRNA) and a CRISPR-associated endonuclease (Cas). The gRNA is meticulously designed to target a specific DNA sequence, guided by a protospacer adjacent motif (PAM) located downstream of the target site. Once the gRNA-Cas complex locates its target within the iPSC genome, the Cas endonuclease induces a double-strand break in the DNA, prompting cellular repair mechanisms to come into play (Jinek et al., 2012).
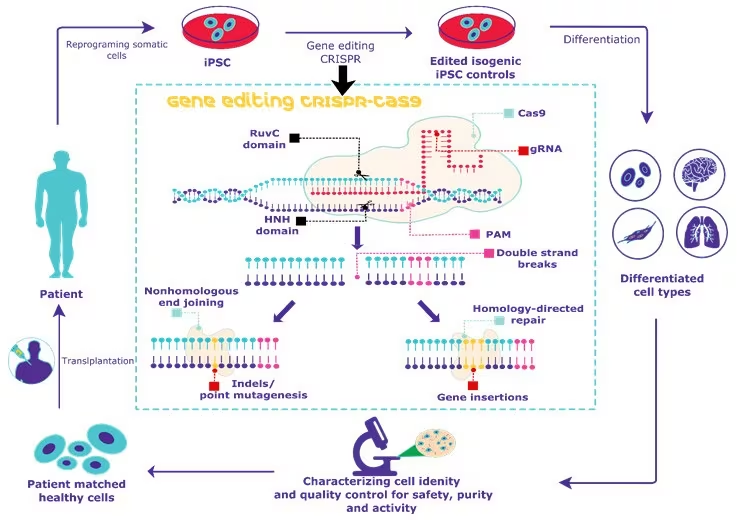
Two primary pathways, non-homologous end joining (NHEJ) and homology-directed repair (HDR), control the DNA repair process in iPSCs. NHEJ often results in error-prone repair, introducing insertions or deletions (indels) that can disrupt gene expression, mainly when they cause frameshift mutations within the iPSCs. In contrast, HDR harnesses a donor DNA template to achieve precise repair or gene insertion, ensuring the desired changes are incorporated into the target DNA (Petazzi et al., 2022). This method enables the generation of knock-outs or knock-ins in iPSCs by replacing or introducing specific gene sequences with unprecedented accuracy and efficiency. The versatility and precision of CRISPR/Cas9 have revolutionised genetic engineering within the framework of iPSCs, offering unparalleled opportunities for advancing iPSC research, personalised medicine applications, and the potential development of targeted therapies for a myriad of genetic disorders (Petazzi et al., 2020).
Challenges and Considerations for iPSC-Based Therapies
While the potential of iPSC technology in translational medicine is evident, significant concerns surrounding reprogramming adult somatic cells must be addressed before its widespread clinical application. The long-term consequences of maintaining an induced pluripotent state remain undetermined, as reprogramming can activate oncogenes and tumour suppressor genes, potentially leading to cancer or teratocarcinoma formation (Laurent et al., 2011). Moreover, the lack of robust and effective differentiation protocols for immature iPSCs poses a significant challenge in therapeutic use (Hamdorf et al., 2015). Successful studies primarily focus on differentiating hematopoietic cells, neurons, or cardiomyocytes, which are comparatively easier to derive from iPSCs. However many other therapeutic cell lines exist, but many available protocols often yield low-efficiency cells with varying potency at maturity. Variability in iPSC neural differentiation, for example, has been observed, with iPSCs displaying lower efficiency than human embryonic stem cells (Hu et al., 2010). This inconsistency persists across reprogramming techniques and multiple iPSC lines, suggesting inherent differences in pluripotent cell lines (Sanchez-Freire et al., 2014). Efforts are underway to enhance differentiation protocols, potentially leveraging epigenetic programming to address the current limitations of iPSC technology.
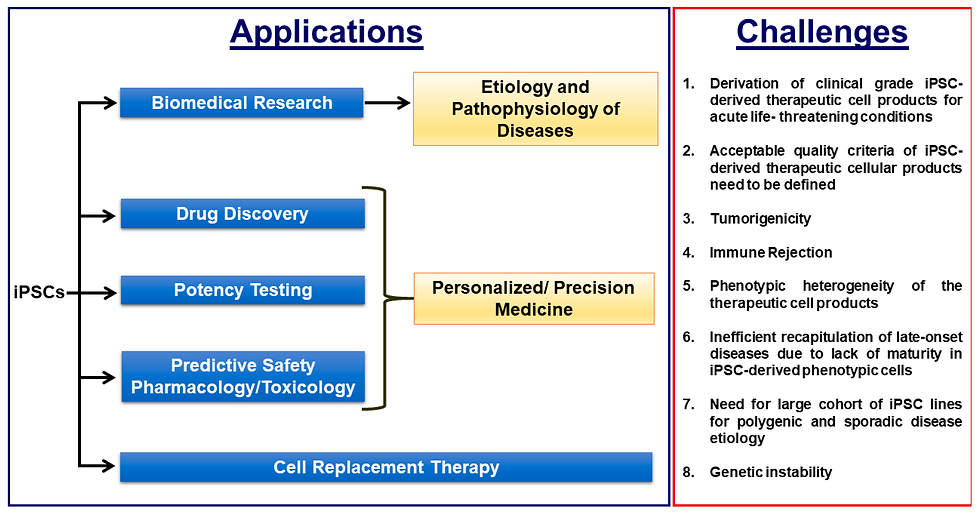
Additionally, advanced genetic analysis has revealed subtle yet significant differences between iPSCs and hESCs, including unique gene expression patterns and variations in DNA methylation. These disparities raise concerns about the safety and efficacy of iPSC-based therapies, particularly regarding the potential for cancer development (Deng et al., 2009). Addressing these challenges and gaining a comprehensive understanding of iPSCs' genetic and chromosomal aberrations during reprogramming, in vitro expansion, and differentiation is essential for their clinical application (Chin et al., 2009). While it is assumed that autologous iPSCs and their derivatives should be immunologically tolerated, the immunogenicity of iPSC derivatives remains a complex and controversial issue. Various factors can contribute to iPSC immunogenicity, including genetic and epigenetic defects during somatic reprogramming, immaturity of in vitro differentiated cells, and culture conditions. Research is ongoing to assess and minimise the risk of immune responses to iPSC-derived therapies, with some studies showing promise in reducing immunogenicity. Understanding and mitigating these risks are crucial steps before iPSCs can be safely applied in clinical settings (Polo et al., 2010; Todorova et al., 2016).
Conclusions
The intersection of iPSCs and genetic engineering signifies a momentous convergence of scientific progress and potential. Nonetheless, as the boundless opportunities are celebrated, the intricate challenges and uncertainties accompanying this undertaking must also be acknowledged. The inquiries surrounding the enduring effects of iPSCs, their differentiation into specialised cell lineages, and immunogenic responses must be meticulously addressed to ensure the safety and efficacy of iPSC-based therapeutic modalities. As the threshold of a new epoch in science and medicine stands, the path ahead is well-defined but contains unknown territories. The comprehension of iPSCs continues to evolve, as does the proficiency of genetic engineering. A collaborative effort, including researchers and clinicians, is indispensable in navigating this course conscientiously and harnessing the full potential of iPSCs and genetic engineering to advance human health. The convergence of iPSCs and genetic engineering epitomises a ray of hope for individuals grappling with genetic disorders and degenerative ailments. It encapsulates the spirit of innovation and the resolute commitment of the scientific community to redefine the boundaries of human knowledge and therapeutics. On the path to discovering effective treatments, prudent optimism is advisable.
Bibliographical References
Benabdellah, K., Sánchez-Hernández, S., Aguilar-González, A., Maldonado-Pérez, N., Gutierrez-Guerrero, A., Cortijo-Gutierrez, M., Ramos-Hernández, I., Tristán-Manzano, M., Galindo-Moreno, P., Herrera, C., & Martin, F. (2020). Genome-edited adult stem cells: Next-generation advanced therapy medicinal products. Stem Cells Translational Medicine, 9(6), 674–685. https://doi.org/10.1002/sctm.19-0338
Chang, C. W., Lai, Y. S., Pawlik, K. M., Liu, K., Sun, C. W., Li, C., Schoeb, T. R., & Townes, T. M. (2009). Polycistronic lentiviral vector for “hit and run” reprogramming of adult skin fibroblasts to induced pluripotent stem cells. Stem Cells, 27(5), 1042–1049. https://doi.org/10.1002/stem.39
Chin, M. H., Mason, M. J., Xie, W., Volinia, S., Singer, M., Peterson, C., Ambartsumyan, G., Aimiuwu, O., Richter, L., Zhang, J., Khvorostov, I., Ott, V., Grunstein, M., Lavon, N., Benvenisty, N., Croce, C. M., Clark, A. T., Baxter, T., Pyle, A. D., … Lowry, W. E. (2009). Induced pluripotent stem cells and embryonic stem cells are distinguished by gene expression signatures. Cell Stem Cell, 5(1), 111–123. https://doi.org/10.1016/J.STEM.2009.06.008
Cole, M. F., Johnstone, S. E., Newman, J. J., Kagey, M. H., & Young, R. A. (2008). Tcf3 is an integral component of the core regulatory circuitry of embryonic stem cells. Genes and Development, 22(6), 746–755. https://doi.org/10.1101/gad.1642408
Deng, J., Shoemaker, R., Xie, B., Gore, A., Leproust, E. M., Antosiewicz-Bourget, J., Egli, D., Maherali, N., Park, I. H., Yu, J., Daley, G. Q., Eggan, K., Hochedlinger, K., Thomson, J., Wang, W., Gao, Y., & Zhang, K. (2009). Targeted bisulfite sequencing reveals changes in DNA methylation associated with nuclear reprogramming. Nature Biotechnology, 27(4), 353–360. https://doi.org/10.1038/nbt.1530
Epsztejn-Litman, S., Feldman, N., Abu-Remaileh, M., Shufaro, Y., Gerson, A., Ueda, J., Deplus, R., Fuks, F., Shinkai, Y., Cedar, H., & Bergman, Y. (2008). De novo DNA methylation promoted by G9a prevents reprogramming of embryonically silenced genes. Nature Structural and Molecular Biology, 15(11), 1176–1183. https://doi.org/10.1038/nsmb.1476
Feng, B., Jiang, J., Kraus, P., Ng, J. H., Heng, J. C. D., Chan, Y. S., Yaw, L. P., Zhang, W., Loh, Y. H., Han, J., Vega, V. B., Cacheux-Rataboul, V., Lim, B., Lufkin, T., & Ng, H. H. (2009). Reprogramming of fibroblasts into induced pluripotent stem cells with orphan nuclear receptor Esrrb. Nature Cell Biology, 11(2), 197–203. https://doi.org/10.1038/ncb1827
Feng, B., Ng, J. H., Heng, J. C. D., & Ng, H. H. (2009). Molecules that promote or enhance reprogramming of somatic cells to induced pluripotent stem cells. Cell Stem Cell, 4(4), 301–312. https://doi.org/10.1016/j.stem.2009.03.005
Frøslev, P., Franzyk, H., Ozgür, B., Brodin, B., & Kristensen, M. (2022). Highly cationic cell-penetrating peptides affect the barrier integrity and facilitates mannitol permeation in a human stem cell-based blood-brain barrier model. European Journal of Pharmaceutical Sciences, 168. https://doi.org/10.1016/j.ejps.2021.106054
Fusaki, N., Ban, H., Nishiyama, A., Saeki, K., & Hasegawa, M. (2009). Efficient induction of transgene-free human pluripotent stem cells using a vector based on Sendai virus, an RNA virus that does not integrate into the host genome. Proceedings of the Japan Academy. Series B, Physical and Biological Sciences, 85(8), 348–362. https://doi.org/10.2183/PJAB.85.348
González, F., Boué, S., & Belmonte, J. C. I. (2011). Methods for making induced pluripotent stem cells: Reprogramming à la carte. Nature Reviews Genetics, 12(4), 231–242. https://doi.org/10.1038/nrg2937
Gurdon, J. B., Elsdale, T. R., & Fischberg, M. (1958). Sexually mature individuals of Xenopus laevis from the transplantation of single somatic nuclei. Nature, 182(4627), 64–65. https://doi.org/10.1038/182064a0
Hamdorf, M., Idica, A., Zisoulis, D. G., Gamelin, L., Martin, C., Sanders, K. J., & Pedersen, I. M. (2015). MiR-128 represses L1 retrotransposition by binding directly to L1 RNA. Nature Structural and Molecular Biology, 22(10), 824–831. https://doi.org/10.1038/nsmb.3090
Hendriks, D., Clevers, H., & Artegiani, B. (2020). CRISPR-Cas tools and their application in genetic engineering of human stem cells and organoids. Cell Stem Cell, 27(5), 705–731 https://doi.org/10.1016/j.stem.2020.10.014
Hu, B. Y., Weick, J. P., Yu, J., Ma, L. X., Zhang, X. Q., Thomson, J. A., & Zhang, S. C. (2010). Neural differentiation of human induced pluripotent stem cells follows developmental principles but with variable potency. Proceedings of the National Academy of Sciences of the United States of America, 107(9), 4335–4340. https://doi.org/10.1073/pnas.0910012107
Hu, W., He, Y., Xiong, Y., Lu, H., Chen, H., Hou, L., Qiu, Z., Fang, Y., & Zhang, S. (2016). Derivation, expansion, and motor neuron differentiation of human-induced pluripotent stem cells with non-integrating episomal vectors and a defined xenogeneic-free culture system. Molecular Neurobiology, 53(3), 1589–1600. https://doi.org/10.1007/s12035-014-9084-z
Ivey, K. N., Muth, A., Arnold, J., King, F. W., Yeh, R. F., Fish, J. E., Hsiao, E. C., Schwartz, R. J., Conklin, B. R., Bernstein, H. S., & Srivastava, D. (2008). MicroRNA regulation of cell lineages in mouse and human embryonic stem cells. Cell Stem Cell, 2(3), 219–229. https://doi.org/10.1016/j.stem.2008.01.016
Jinek, M., Chylinski, K., Fonfara, I., Hauer, M., Doudna, J. A., & Charpentier, E. (2012). A programmable dual-RNA-guided DNA endonuclease in adaptive bacterial immunity. Science, 337(6096), 816–821. https://doi.org/10.1126/science.1225829
Kim, D., Kim, C. H., Moon, J. Il, Chung, Y. G., Chang, M. Y., Han, B. S., Ko, S., Yang, E., Cha, K. Y., Lanza, R., & Kim, K. S. (2009). Generation of human induced pluripotent stem cells by direct delivery of reprogramming proteins. Cell Stem Cell, 4(6), 472–476. https://doi.org/10.1016/j.stem.2009.05.005
Laurent, L. C., Ulitsky, I., Slavin, I., Tran, H., Schork, A., Morey, R., Lynch, C., Harness, J. V., Lee, S., Barrero, M. J., Ku, S., Martynova, M., Semechkin, R., Galat, V., Gottesfeld, J., Belmonte, J. C. I., Murry, C., Keirstead, H. S., Park, H. S., … Loring, J. F. (2011). Dynamic changes in the copy number of pluripotency and cell proliferation genes in human ESCs and iPSCs during reprogramming and time in culture. Cell Stem Cell, 8(1), 106–118. https://doi.org/10.1016/j.stem.2010.12.003
Lee, M., Seok, J., Saha, S. K., Cho, S., Jeong, Y., Gil, M., Kim, A., Shin, H. Y., Bae, H., Do, J. T., Kim, Y. B., & Cho, S. G. (2023). Alterations and co-occurrence of C-MYC, N-MYC, and L-MYC expression are related to clinical outcomes in various cancers. International Journal of Stem Cells, 16(2), 215–233. https://doi.org/10.15283/ijsc22188
Leo, S., Kato, Y., Wu, Y., Yokota, M., Koike, M., Yui, S., Tsuchiya, K., Shiraki, N., & Kume, S. (2023). The effect of vitamin D3 and valproic acid on the maturation of human-induced pluripotent stem cell-derived enterocyte-like cells. Stem Cells, 41(8). https://doi.org/10.1093/stmcls/sxad042
Liang, J., Wan, M., Zhang, Y., Gu, P., Xin, H., Jung, S. Y., Qin, J., Wong, J., Cooney, A. J., Liu, D., & Songyang, Z. (2008). Nanog and Oct4 associate with unique transcriptional repression complexes in embryonic stem cells. Nature Cell Biology, 10(6), 731–739. https://doi.org/10.1038/ncb1736
Lin, S. L., Chen, J. S., & Ying, S. Y. (2020). MiR-302-mediated somatic cell reprogramming and method for generating tumor-free iPS cells using miR-302. Methods in Molecular Biology, 2115, 199–219. https://doi.org/10.1007/978-1-0716-0290-4_12
Liu, C., Carrera, R., Flamini, V., Kenny, L., Cabahug-Zuckerman, P., George, B. M., Hunter, D., Liu, B., Singh, G., Leucht, P., Mann, K. A., Helms, J. A., & Castillo, A. B. (2018). Effects of mechanical loading on cortical defect repair using a novel mechanobiological model of bone healing. Bone, 108, 145–155. https://doi.org/10.1016/j.bone.2017.12.027
Liu, J., & Verma, P. J. (2015). Synthetic mRNA reprogramming of human fibroblast cells. Methods in Molecular Biology, 1330, 17–28. https://doi.org/10.1007/978-1-4939-2848-4_2
Liu, S. P., Fu, R. H., Huang, S. J., Huang, Y. C., Chen, S. Y., Chang, C. H., Liu, C. H., Tsai, C. H., Shyu, W. C., & Lin, S. Z. (2013). Stem cell applications in regenerative medicine for neurological disorders. Cell Transplantation, 22(4), 631–637. https://doi.org/10.3727/096368912X655145
Mali, P., Ye, Z., Hommond, H. H., Yu, X., Lin, J., Chen, G., Zou, J., & Cheng, L. (2008). Improved efficiency and pace of generating induced pluripotent stem cells from human adult and fetal fibroblasts. Stem Cells, 26(8), 1998–2005. https://doi.org/10.1634/stemcells.2008-0346
Markoulaki, S., Hanna, J., Beard, C., Carey, B. W., Cheng, A. W., Lengner, C. J., Dausman, J. A., Fu, D., Gao, Q., Wu, S., Cassady, J. P., & Jaenisch, R. (2009). Transgenic mice with defined combinations of drug-inducible reprogramming factors. Nature Biotechnology, 27(2), 169–171. https://doi.org/10.1038/nbt.1520
Menon, S., Shailendra, S., Renda, A., Longaker, M., & Quarto, N. (2016). An overview of direct somatic reprogramming: The ins and outs of iPSCs. International Journal of Molecular Sciences, 17(1), 1–20. https://doi.org/10.3390/ijms17010141
Meshorer, E., & Misteli, T. (2006). Chromatin in pluripotent embryonic stem cells and differentiation. Nature Reviews Molecular Cell Biology, 7(7), 540–546. https://doi.org/10.1038/nrm1938
Mikkelsen, T. S., Hanna, J., Zhang, X., Ku, M., Wernig, M., Schorderet, P., Bernstein, B. E., Jaenisch, R., Lander, E. S., & Meissner, A. (2008). Dissecting direct reprogramming through integrative genomic analysis. Nature, 454(7200), 49–55. https://doi.org/10.1038/nature07056
Nakagawa, M., Koyanagi, M., Tanabe, K., Takahashi, K., Ichisaka, T., Aoi, T., Okita, K., Mochiduki, Y., Takizawa, N., & Yamanaka, S. (2008). Generation of induced pluripotent stem cells without Myc from mouse and human fibroblasts. Nature Biotechnology, 26(1), 101–106. https://doi.org/10.1038/nbt1374
Niwa, H. (2007). How is pluripotency determined and maintained? Development, 134(4), 635–646. https://doi.org/10.1242/dev.02787
Park, I. H., Zhao, R., West, J. A., Yabuuchi, A., Huo, H., Ince, T. A., Lerou, P. H., Lensch, M. W., & Daley, G. Q. (2008). Reprogramming of human somatic cells to pluripotency with defined factors. Nature, 451(7175), 141–146. https://doi.org/10.1038/nature06534
Petazzi, P., Menéndez, P., & Sevilla, A. (2022). CRISPR/Cas9–mediated gene knockout and knockin human iPSCs. Methods in Molecular Biology, 2454, 559–574. https://doi.org/10.1007/7651_2020_337
Petazzi, P., Torres-Ruiz, R., Fidanza, A., Roca-Ho, H., Gutierrez-Agüera, F., Castaño, J., Rodriguez-Perales, S., Díaz de la Guardia, R., López-Millán, B., Bigas, A., Forrester, L. M., Bueno, C., & Menéndez, P. (2020). Robustness of catalytically dead cas9 activators in human pluripotent and mesenchymal stem cells. Molecular Therapy - Nucleic Acids, 20, 196–204. https://doi.org/10.1016/j.omtn.2020.02.009
Polo, J. M., Liu, S., Figueroa, M. E., Kulalert, W., Eminli, S., Tan, K. Y., Apostolou, E., Stadtfeld, M., Li, Y., Shioda, T., Natesan, S., Wagers, A. J., Melnick, A., Evans, T., & Hochedlinger, K. (2010). Cell type of origin influences the molecular and functional properties of mouse induced pluripotent stem cells. Nature Biotechnology, 28(8), 848–855. https://doi.org/10.1038/nbt.1667
Sanchez-Freire, V., Lee, A. S., Hu, S., Abilez, O. J., Liang, P., Lan, F., Huber, B. C., Ong, S. G., Hong, W. X., Huang, M., & Wu, J. C. (2014). Effect of human donor cell source on differentiation and function of cardiac induced pluripotent stem cells. Journal of the American College of Cardiology, 64(5), 436–448. https://doi.org/10.1016/j.jacc.2014.04.056
Sarig, R., Rivlin, N., Brosh, R., Bornstein, C., Kamer, I., Ezra, O., Molchadsky, A., Goldfinger, N., Brenner, O., & Rotter, V. (2010). Mutant p53 facilitates somatic cell reprogramming and augments the malignant potential of reprogrammed cells. Journal of Experimental Medicine, 207(10), 2127–2140. https://doi.org/10.1084/jem.20100797
Sellers, Z. P., Schneider, G., Maj, M., & Ratajczak, M. Z. (2018). Analysis of the paternally-imprinted DLK1–MEG3 and IGF2–H19 tandem gene loci in NT2 embryonal carcinoma cells identifies DLK1 as a potential therapeutic target. Stem Cell Reviews and Reports, 14(6), 823–836. https://doi.org/10.1007/s12015-018-9838-5
Shi, Y., Do, J. T., Desponts, C., Hahm, H. S., Schöler, H. R., & Ding, S. (2008). A combined chemical and genetic approach for the generation of induced pluripotent stem cells. Cell Stem Cell, 2(6), 525–528. https://doi.org/10.1016/j.stem.2008.05.011
Silva, J., & Smith, A. (2008). Capturing pluripotency. Cell, 132(4), 532–536. https://doi.org/10.1016/j.cell.2008.02.006
Sridharan, R., Tchieu, J., Mason, M. J., Yachechko, R., Kuoy, E., Horvath, S., Zhou, Q., & Plath, K. (2009). Role of the murine reprogramming factors in the induction of pluripotency. Cell, 136(2), 364–377. https://doi.org/10.1016/j.cell.2009.01.001
Takahashi, K., Tanabe, K., Ohnuki, M., Narita, M., Ichisaka, T., Tomoda, K., & Yamanaka, S. (2007). Induction of pluripotent stem cells from adult human fibroblasts by defined factors. Cell, 131(5), 861–872. https://doi.org/10.1016/j.cell.2007.11.019
Takahashi, K., & Yamanaka, S. (2006). Induction of pluripotent stem cells from mouse embryonic and adult fibroblast cultures by defined factors. Cell, 126(4), 663–676. https://doi.org/10.1016/j.cell.2006.07.024
Tasca, F., Wang, Q., & Gonçalves, M. A. F. V. (2020). Adenoviral vectors meet gene editing: A rising partnership for the genomic engineering of human stem cells and their progeny. Cells, 9(4). https://doi.org/10.3390/cells9040953
NobelPrize.org (2012). The Nobel Prize in Physiology or Medicine 2012. Retrieved September 16, 2023, from https://www.nobelprize.org/prizes/medicine/2012/yamanaka/biographical/
Todorova, D., Kim, J., Hamzeinejad, S., He, J., & Xu, Y. (2016). Brief report: Immune microenvironment determines the immunogenicity of induced pluripotent stem cell derivatives. Stem Cells, 34(2), 510–515. https://doi.org/10.1002/stem.2227
Warren, L., Manos, P. D., Ahfeldt, T., Loh, Y. H., Li, H., Lau, F., Ebina, W., Mandal, P. K., Smith, Z. D., Meissner, A., Daley, G. Q., Brack, A. S., Collins, J. J., Cowan, C., Schlaeger, T. M., & Rossi, D. J. (2010). Highly efficient reprogramming to pluripotency and directed differentiation of human cells with synthetic modified mRNA. Cell Stem Cell, 7(5), 618–630. https://doi.org/10.1016/j.stem.2010.08.012
Wernig, M., Meissner, A., Cassady, J. P., & Jaenisch, R. (2008). c-Myc is dispensable for direct reprogramming of mouse fibroblasts. Cell Stem Cell, 2(1), 10–12. https://doi.org/10.1016/j.stem.2007.12.001
Woodard, L. E., & Wilson, M. H. (2015). PiggyBac-ing models and new therapeutic strategies. Trends in Biotechnology, 33(9), 525–533. https://doi.org/10.1016/j.tibtech.2015.06.009
Wu, J., & Izpisua Belmonte, J. C. (2015). Dynamic pluripotent stem cell states and their applications. Cell Stem Cell, 17(5), 509–525. https://doi.org/10.1016/j.stem.2015.10.009
Yu, J., & Thomson, J. A. (2008). Pluripotent stem cell lines. Genes and Development, 22(15), 1987–1997. https://doi.org/10.1101/gad.1689808
Zeng, J., Li, Y., Ma, Z., & Hu, M. (2020). Advances in small molecules in cellular reprogramming: Effects, structures, and mechanisms. Current Stem Cell Research & Therapy, 16(2), 115–132. https://doi.org/10.2174/1574888x15666200621172042
Zhou, W., & Freed, C. R. (2009). Adenoviral gene delivery can reprogram human fibroblasts to induced pluripotent stem cells. Stem Cells, 27(11), 2667–2674. https://doi.org/10.1002/stem.201
Visual Sources
Cover Image: Wüstefeld, T. (2021). An illustration of single-cell RNA sequencing. [Illustration]. https://www.embl.org/news/science/ipscs-and-disease/
Figure 1: Historical advancements in iPSCs research. (2016). [Infographic]. Gladstone Institute. https://gladstone.org/
Figure 2: Applications of iPSCs in medicine. (2023). [Illustration]. Atlantis Bioscience. https://www.atlantisbioscience.com.sg/revolutionising-medicine-the-power-of-induced-pluripotent-stem-cells-ipscs/
Figure 3: The main steps of iPSCs generation. Bailly, A., Milhavet, O., & Lemaitre, J. M. (2022). [Illustration]. RNA-based strategies for cell reprogramming toward pluripotency. Pharmaceutics, 14(2), 317. Multidisciplinary Digital Publishing Institute. https://doi.org/10.3390/pharmaceutics1020317
Figure 4: The interchangeability of OSKM with other factors. Heng, J. C. D., Feng, B., Han, J., Jiang, J., Kraus, P., Ng, J. H., Orlov, Y. L., Huss, M., Yang, L., Lufkin, T., Lim, B., & Ng, H. H. (2010). [Illustration]. The nuclear receptor Nr5a2 can replace Oct4 in the reprogramming of murine somatic cells to pluripotent cells. Cell Stem Cell, 6(2), 167–174. https://doi.org/10.1016/j.stem.2009.12.009
Figure 5: Principles of retroviral vector gene transfer. Jain, S., Yadav, M. K., & Kumar, A. (2018). [Illustration]. Techniques for production and quality assessment of genetically modified foods. In Holban, A., M., & Grumezescu, A., M. (Eds.) Genetically Engineered Foods (Vol. 6, pp. 177–202). Academic Press. https://doi.org/10.1016/B978-0-12-811519-0.00007-8
Figure 6: The mechanism of piggyBac transposition. Woodard, L. E., & Wilson, M. H. (2015). [Illustration]. PiggyBac-ing models and new therapeutic strategies. Trends in Biotechnology, 33(9), 525–533. https://doi.org/10.1016/j.tibtech.2015.06.009
Figure 7: Overview of cellular reprogramming into iPSCs using non-transmissible Sendai Virus vectors. Bonaventura, Gabriele & Barcellona, Maria & Iemmolo, Rosario & Cavallaro, Sebastiano. (2017). [Illustration]. Induced pluripotent stem cells: An innovative patient-specific neurodegenerative disease modeling. Journal of Stem Cell and Transplantation Biology. 2(2), 112. Research Gate.
Figure 8: Cellular uptake mechanism of cell-penetrating peptides (CPP)-conjugated proteins. Seo, B. J., Hong, Y. J., & Do, J. T. (2017). [Illustration]. Cellular reprogramming using protein and cell-penetrating peptides. International Journal of Molecular Sciences, 18(3), 552. Multidisciplinary Digital Publishing Institute. https://doi.org/10.3390/ijms18030552
Figure 9: Somatic cells can be reprogrammed by using the transcription factors OCT-4, SOX-2, KLF-4, and MYC or by adding miRNAs alone. (n.d.). [Illustration]. Creative Bioarray. https://www.creative-bioarray.com/support/ipsc-generation-using-microrna-mir-302-based-reprogramming-method.htm
Figure 10: Regulation of reprogramming and iPSC differentiation by epigenetic mechanisms. Huang, C., & Wu, J. C. (2012). [Illustration]. Epigenetic modulations of induced pluripotent stem cells: Novel therapies and disease models. Drug Discovery Today: Disease Models, 9(4), 153-160. https://doi.org/10.1016/j.ddmod.2012.02.004
Figure 11: The pluripotency and self-renewal transcriptional network. Cambuli, Francesco. (2015). [Illustration]. ES cell transdifferentiation to TS-like cells. [Doctoral dissertation, University of Cambridge]. Research Gate.
https://www.researchgate.net/publication/278966289_ES_cell_transdifferentiation_to_TS-like_cells
Figure 12: Overview of CRISPR gene editing of human iPSCs. (n.d.). [Illustration]. Sigma Aldrich. https://www.sigmaaldrich.com/DE/de/technical-documents/technical-article/genomics/advanced-gene-editing/human-ipsc-crispr-protocol
Figure 13: Biomedical applications of iPSCs and the critical challenges that need to be overcome for efficient clinical translation. Doss, M. X., & Sachinidis, A. (2019). [Illustration]. Current challenges of iPSC-based disease modeling and therapeutic implications. Cells, 8(5), 403. Cells. https://doi.org/10.3390/cells8050403
Comments