The Evolution of Polymers
- Nicole Galetti
- Dec 17, 2023
- 8 min read
Updated: Jan 27, 2024
The term polymer is derived from the Greek words "polus", which means many or much, and "meros", which means parts. So, it can be translated as “many parts”, which is exactly what polymers are: they are constructed and consist of many repeating parts. A polymer is a material or substance that consists of larger molecules, known as macromolecules or monomers, that are added in a repeating structural pattern (Wypych, 2022). Polymers have evolved through a fascinating journey through technological advancements and scientific breakthroughs. This field has burgeoned from humble beginnings to become an integral aspect of modern materials science, revolutionizing industries ranging from packaging and textiles to medicine and electronics. Polymers range from synthetic materials such as polystyrene to biological polymers such as DNA and proteins. Both natural and synthetic polymers are synthesized via the process known as polymerization (Feldman, 2008). There are a few physical and chemical properties that stand out for polymeric materials; they usually have large molecular masses, high elasticity, high viscoelasticity, and a tendency to form amorphous or semicrystalline structures.
Historical Perspective of Polymers
From the dawn of civilization, polymers have been critical components of commodities. Keratin used for wool, cellulose used in cotton, and linen fibres for garments, as well as paper, are just a few examples of how ancient societies exploited raw materials containing polymer structures. The Olmec, Maya, and Aztec civilizations from South America used the latex sap of "caoutchouc" trees (natural rubber) to make balls, waterproof textiles, and containers by the 16th century [Figure 1]. Even though there was a lack of theoretical knowledge, the industrial aspect of polymers to provide accessible and affordable materials to the world was immediately recognised. Significant advances in the field of polymer science were brought forward by Henri Braconnot, Friedrich Ludersdorf, Nathaniel Hayward, Alexander Parkes and Charles Goodyear, mostly by the modifications of natural polymers (Feldman, 2008; Krauklis et al., 2022). They discovered materials such as celluloid, galalith, parkesine, rayon, vulcanized rubber, and later on bakelite. These materials quickly entered the industrial market and reached households as fabrics, buttons, crockery and decorative items.
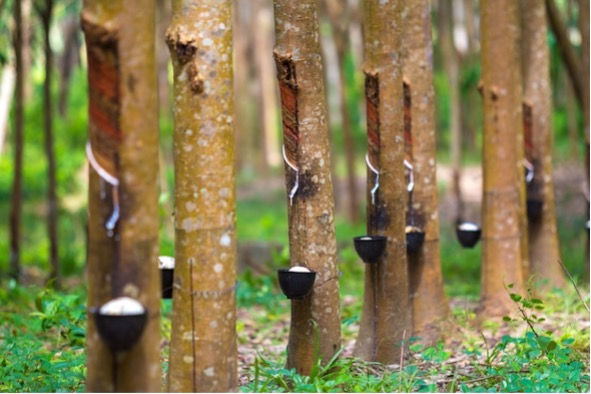
Hermann Staudinger [Figure 2] proposed the modern definition of polymers as covalently bonded macromolecules that are long chains of atoms linked together in 1920. This led him to spend his whole career defining this definition experimentally and because of his work, Staudinger was awarded the Nobel Prize in chemistry in 1953 (Ciolino et al., 2022). The primary focus of polymer science has historically been the discovery of products from the chemical linkage of repeating units by covalent bonding. In recent decades, advanced polymer technologies have emerged, including biodegradable polymers, conducting polymers, and smart polymers that respond to external stimuli. The integration of polymers into nanotechnology further expanded their utility, opening avenues for novel applications in fields like drug delivery, tissue engineering, and electronics. In this evolutionary journey, the synthesis techniques, characterization methods, and applications of polymers have evolved hand in hand, reflecting the interdisciplinary nature of polymer science. The continuous exploration of new monomers, polymerization methods, and sustainable practices underscores the dynamic nature of this field, poised to address contemporary challenges such as environmental sustainability and resource conservation.
This introduction merely scratches the surface of the rich history and multifaceted evolution of polymers. From their humble beginnings as natural substances to the highly engineered materials of today, polymers epitomize the ingenuity and adaptability of scientific inquiry, promising a future filled with innovative solutions and unprecedented possibilities.
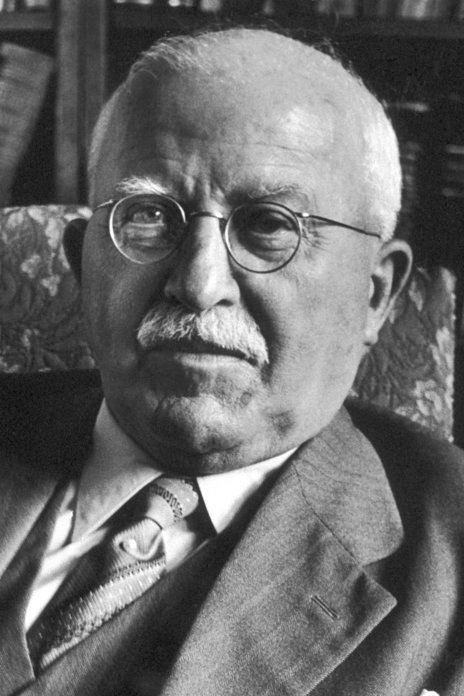
Polymers and their Applications
There are several types of polymers, some of which are mentioned in the introduction of this article. Among the main types of polymers are synthetic, natural, supramolecular, addition, condensation and rearrangement (Wahby, 2003; Wypych, 2022). The advent of synthetic polymers during the mid-20th century began with Wallace Carothers at DuPont for developing nylon in the 1930s, followed by the explosion of polymer research post-World War II. This era saw the emergence of prominent polymers such as polyester, polyethylene, and polypropylene, laying the foundation for a plethora of applications across various industries. According to global demand, the list of synthetic polymers, in order, includes the following: Polyethylene, polypropylene, polystyrene, polyvinylchloride, synthetic rubber (petroleum-based monomers), bakelite (phenol formaldehyde resin), neoprene, nylon, polyacrylonitrile, polyvinyl butyral (PVB), silicone among others.
Supramolecular polymers [Figure 3] with non-covalent linkages have been a new and exciting area of research for polymer scientists (Gourmelon, 2015). Some of the applications of supramolecular polymers include the biomedical field, for example, smart drug delivery systems, self-healing materials and tissue engineering. Latex rubber, otherwise known as polyisoprene, is an example of a natural polymer along with cellulose, which is the main constituent of wood and paper. However, a majority of these natural polymeric macromolecules are found in biological systems. These include proteins (polyamides), nucleic acids (polynucleotides), and polysaccharides. Other natural polymeric materials such as hemp, shellac, amber, wool, and silk have been used for centuries.
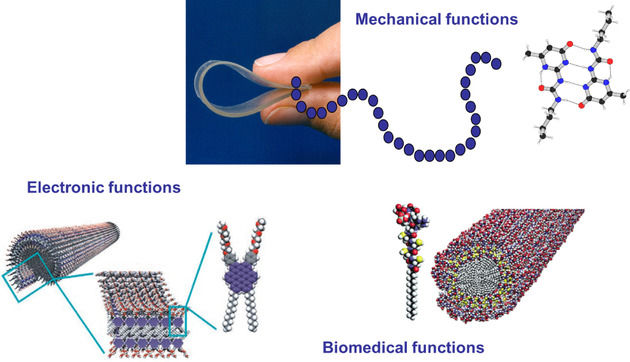
Nowadays, synthetic polymers have become integral to virtually every aspect of our daily lives. The absence of these polymers would drastically alter the landscape of modern society. The widespread adoption of polymers can be attributed to their distinctive attributes, including low density, cost-effectiveness, excellent thermal/electrical insulation properties, high resistance to corrosion, energy-efficient manufacturing processes, and easy transformation into finished products. The unique properties of polymers can be fine-tuned or augmented for specific applications through combinations with other materials, such as in composites with nanomaterials, polysaccharides, chitosan and nanotubes. The utilization of polymers brings about significant advantages, contributing to energy conservation (e.g., in the production of lighter cars and planes no need for a comma when there are two items and thermally insulated buildings), safeguarding food and drinking water (through packaging), reducing land usage and fertilizer consumption (in the production of synthetic fibres), preserving materials (coatings), and protecting and enhancing human lives in fields like hygiene and medical applications (Brady et al., 2017; Gruber & Kunz, 1971).
Polymer Structures and Synthesis
Taking a look at the structure of polymers, a few commonalities can be found between them. First of all, the continuously linked backbone of a polymer consists mainly of carbon atoms. An example of this is polyethylene, whose structure consists of repeating units of the monomer ethylene [Figure 4]. Other structures also exist, for example, silicon or polysiloxane, which are part of the silicon family of polymers and consist mainly of repeating units of siloxane. These polymers are being used in putty and waterproof sealants to name a few [Figure 4]. Polyethylene glycol, polysaccharides and DNA all contain oxygen in their polymer backbones in the form of ether, glucosidic and phosphodiester bonds (Figure 3).
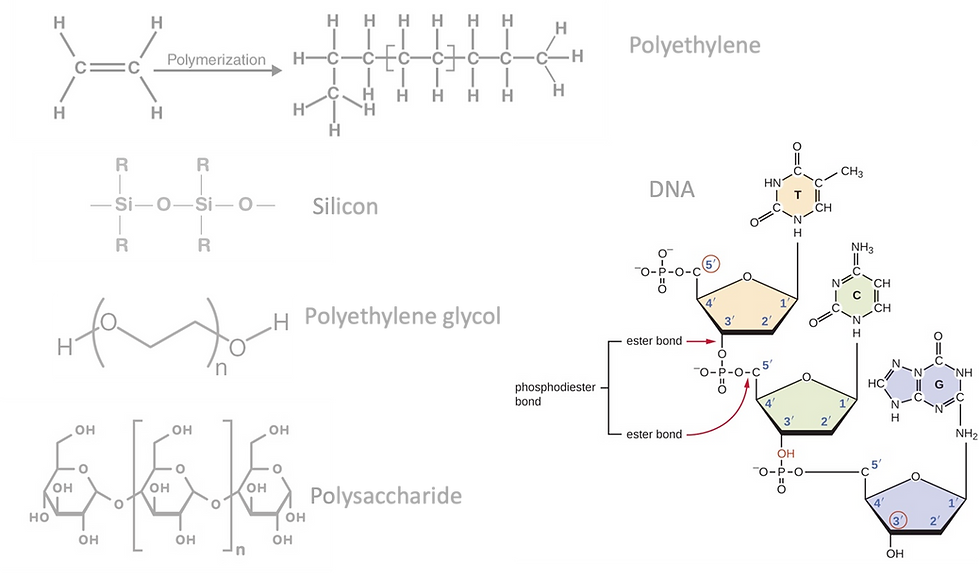
There are several types of polymerization reactions, however in the end all polymers consist of repeating units of monomers. The synthesis of polymers can generally be divided into two categories: chain polymerization and step-growth polymerization. During chain polymerization, the addition of monomers to the growing polymer proceeds “one at a time”. An example of where this is done is the synthesis of polystyrene. During step-growth polymerization, chains of monomers are combined with one another directly, for example, polyester (Yokozawa & Ohta, 2016). Step-growth polymerization can be further divided into polymerization by condensation or polymerization by addition. During polycondensation, low molecular mass by-products are formed in every reaction step, and during polyaddition, a polymer is formed by chain addition reactions between monomers that contain a double bond. For example, the polymerization of PET is the condensation reaction of ethylene glycol and terephthalic acid. Ethylene glycol is a colourless liquid obtained from ethylene, and terephthalic acid is a crystalline solid obtained from xylene. During the reaction, two molecules of water are lost [Figure 5]. Polystyrene on the other hand is an example of an addition reaction. These styrene monomers contain vinyl groups known as double bonds. Two styrene molecules react with one another, and the double bond from a styrene molecule is broken allowing the formation of a new single bond between the two.
There are several classes of chain-growth polymerization, some of which include radical polymerization, ionic polymerization, coordination polymerization, living polymerization, and several more. Some of the most common materials used today are produced through chain-growth polymerization. Some examples of these are polyethylene (PE), polypropylene (PP), polyvinylchloride (PVC), and polyvinyl acetate (PVA). There are three distinct reaction steps or phases that occur in chain-growth polymerization: the initiation phase, the chain propagation phase and finally the chain termination phase. In all chain-growth polymerization reactions, chain initiation and chain propagation phases are present. However, in many reactions chain termination phase may or may not be present. An example of radical free chain-growth polymerization includes the synthesis of polyvinyl acetate. Plasma polymerization is a newer method that does not neatly fit into either chain growth or step-growth polymerization category. The synthesis of biopolymers, especially proteins, is another active area of research (Colombani, 1997; Yokozawa & Ohta, 2016).
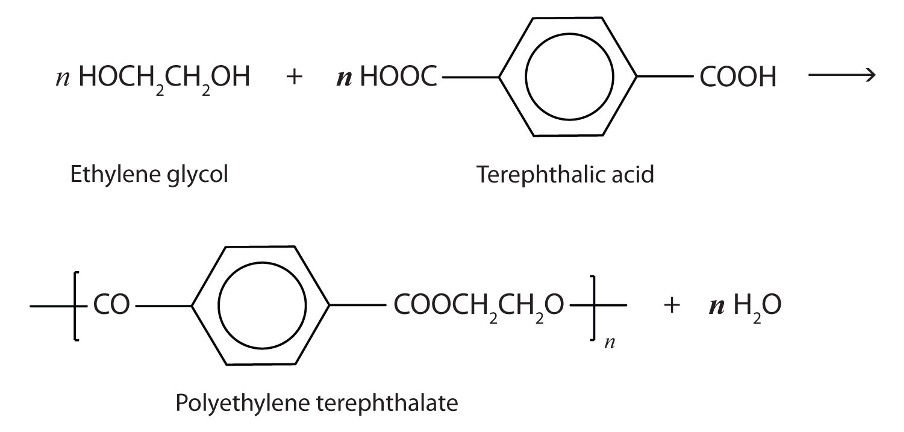
There are three main classes of natural biopolymers: polypeptides, polysaccharides and polynucleotides. In cells, these are synthesized via enzyme-mediated processes. For example, DNA is synthesized by the enzyme DNA polymerase. Proteins are synthesized from amino acids via multiple enzyme-mediated processes. Once the protein is synthesized, it may be further modified to provide the appropriate structure and function. Other types of biopolymers include synthetic biopolymers such as rubber, suberin, melanin, and lignin. Prior to the introduction of synthetic polymers like polyethene and perspex, naturally occurring polymers such as cotton, starch, and rubber were common. Numerous polymers of significant commercial value are produced through the chemical alteration of naturally existing polymers. Notable instances involve the combination of nitric acid with cellulose, resulting in the creation of nitrocellulose, and the process of vulcanizing rubber through the application of heat and sulfur to natural rubber. Various methods for polymer modification encompass oxidation, cross-linking, and end-capping (Van de Velde & Kiekens, 2002).
Polymer Degradation
Several environmental factors, such as heat, light, certain chemicals, oxygen, and enzymes, can contribute to the degradation of certain polymers and ultimately affect properties such as tensile strength, colour, shape, and molecular weight of the polymers. It is believed that this type of degradation is due to bond-bond breaking or chain scission, which occurs in random locations in the polymer backbone [Figure 6]. It is common for these changes to be undesirable, but in some cases, such as biodegradation and recycling, they may prevent pollution of the environment.
Degradation has also proved advantageous in the biomedical field. As an example, hydrolysable stitches are made from a copolymer of polylactic acid and polyglycolic acid, that slowly dissolve after being paced to stitch a wound. The chemical structure of a polymer contributes to its susceptibility to degradation. Generally aromatic and epoxide-containing polymers are more susceptible to UV degradation while polyesters can be degraded by the means of hydrophilic processing (deliberately placing hydroxyl and carboxyl end groups), promoting its water-adsorbing capacity and therefore degradability. Polymers containing double-bond moieties in their backbone can be degraded via ozone cracking, which essentially breaks those double bonds and the structure of the polymer. In most high-temperature applications, carbon-based polymers are not as suitable as they are more susceptible to thermal degradation in comparison to inorganic polymers such as polydimethylsiloxane. For example, as polyethylene degrades, the bonds holding the atoms of the polymer together break at random temperatures above 450 °C, which results in the formation of a mixture of hydrocarbons. In some cases of chain-end scission, monomers are released and this process is referred to as unzipping or depolymerization. The resulting degradation mechanism depends on the type of polymer as well as the applied temperature. Generally, polymers will decompose to small single substituents via random chain breaking. Polymer waste can be sorted for recycling purposes using resin identification codes developed by the Society of the Plastics Industry (Vohlídal, 2020).
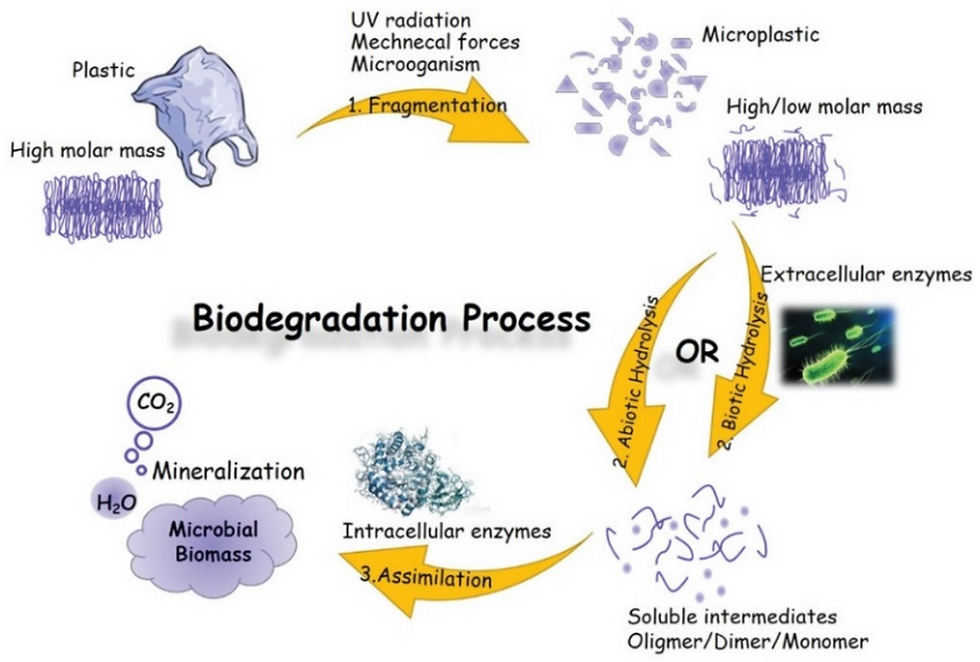
Conclusion
Polymers have evolved in a remarkable way, significantly altering the landscape of materials science and everyday life. From the early discovery of natural polymers to the synthesis of innovative synthetic polymers, this progression has revolutionized industries, technology, and countless aspects of our daily existence. Due to the continuous developments in polymer science, new materials with tailored properties have been developed, opening up new applications. The use of advanced polymers has found its way into various industries such as healthcare, electronics, packaging, and transportation by providing solutions to challenges and increasing efficiency. As a result of increased environmental consciousness, biodegradable and biobased polymers are now being explored, aiming to reduce traditional polymer's ecological footprint. This reflects a growing awareness of the importance of mitigating environmental impact and promoting a circular economy.
Research and innovation will continue to shape polymer evolution as we further address emerging challenges and push the boundaries of material science. Research in polymers will continue to be interdisciplinary by incorporating chemistry, physics, engineering, and biology, paving the way for new applications in the field. The evolution of polymers is ultimately a story of adaptability, ingenuity, and transformation. The journey from natural polymers to modern synthetic materials demonstrates how scientific discovery has shaped our world and improved our quality of life.
Bibliographical References
Aida, T., & Meijer, E. W. (2020). Supramolecular Polymers – we've Come Full Circle. Israel Journal of Chemistry, 60(1-2), 33–47. https://doi.org/https://doi.org/10.1002/ijch.201900165
Brady, J., Dürig, T., Lee, P., & Li, J.-X. (2017). Polymer properties and characterization. Developing Solid Oral Dosage Forms (pp. 181-223). Elsevier.
Ciolino, A. E., de Freitas, A. G., Satti, A. J., & Ninago, M. D. (2022). From atoms to macromolecules: 100 Years of polymer research. Advances in Organic Synthesis, 16, 212.
Colombani, D. (1997). Chain-growth control in free radical polymerization. Progress in Polymer Science, 22(8), 1649–1720. https://doi.org/10.1016/S0079-6700(97)00022-1
Feldman, D. (2008). Polymer history. Designed Monomers and Polymers, 11(1), 1–15. https://doi.org/10.1163/156855508X292383
Gourmelon, G. (2015). Global plastic production rises, recycling lags. Vital Signs, 22, 91–95.
Gruber, C., & Kunz, H. (1971). General properties of polymer systems. Springer.
Krauklis, A. E., Karl, C. W., Rocha, I. B. C. M., Burlakovs, J., Ozola-Davidane, R., Gagani, A. I., & Starkova, O. (2022). Modelling of environmental ageing of polymers and polymer composites; modular and multiscale methods. Polymers, 14(1), 216. https://www.mdpi.com/2073-4360/14/1/216
Van de Velde, K., & Kiekens, P. (2002). Biopolymers: overview of several properties and consequences on their applications. Polymer testing, 21(4), 433–442. https://doi.org/10.1016/S0142-9418(01)00107-6
Vohlídal, J. (2020). Polymer degradation: A short review. Chemistry Teacher International, 3(2), 213–220. https://doi.org/10.1515/cti-2020-0015
Wahby, W. (2003). Fifty years' history of polymers in concrete in review. Special Publication, 214, 13–22. https://doi.org/10.14359/12757
Wypych, G. (2022). Handbook of polymers. Elsevier.
Yokozawa, T., & Ohta, Y. (2016). Transformation of step-growth polymerization into living chain-growth polymerization. Chemical Reviews, 116(4), 1950–1968. https://doi.org/10.1021/acs.chemrev.5b00393
I have understood the principles of Polymers through this article. I always find a lot of useful knowledge from geometry dash meltdown or this website.